Study Objectives
- To define the concepts gap junction, motor unit, synaptic &
neuromuscular transfer, isometric and isotonic contraction, plasticity,
post-synaptic potentials, and recruitment.
- To describe the electromyogram, three types of motor units and three
types of muscle tissue (striated, smooth, and myocardial tissue), modulation
of neurotransmission with facilitation, potentiation, neurotransmitters and
receptors.
- To explain the function of the neuromuscular junction, the synapses,
the neurotransmitters, and the control of the muscular force by frequency
variation and recruitment. To explain disorders of the neuromuscular junction,
the skeletal muscles, the smooth muscles and the myocardium.
- To use the above concepts in problem solving.
Principles
- Waller’s law of neuronal degeneration: When a motor axon has been severed,
the rough endoplasmic reticulum accumulates proteins required for repair of
the axon. The axon and the myelin sheath distal to the injury die and are
phagocytized. The neuroglial Schwann cells remain alive, proliferate and form
long rows along the pathway previously occupied by the dead axon. The severed
axon regenerate along this pathway.
- Dale’s law: A single neuron liberates only one neurotransmitter at all its
synapses. Although the law is frequently valid, there are several exceptions,
where two or more co-transmitters are released at all the synapses of a single
neuron.
Definitions
- Excitatory postsynaptic potential
(EPSP) refers to a
transientdepolarization of a neuron membrane. The combined effect of EPSPs
from hundreds of presynaptic terminals can summate to evoke an action
potential.
Gap junctions are transmembrane protein pores between cells. The pores
represent a low electrical resistance. Most electrical synapses contain
many gap junctions allowing free passage of ions and small molecules in both
directions when open.
Inhibitory postsynaptic potential (IPSP) is a transient
hyperpolarization of a neuron membrane. The negativity of the resting membrane
potential increases (normally -70 mV) and summation of IPSPs may result in an
effect.
Isometric contraction is a muscular contraction at constant length.
Isotonic contraction is a muscle contraction at constant tension (load).
A miniature endplate potential is probably caused by the spontaneous
release of a single acetylcholine vesicle into the synaptic cleft. This is
called quantal release.
Motor unit refers to one motor neuron and the group of muscle fibres it
innervates. All muscle fibres belonging to a certain motor unit are of the same
type.
Neurotransmission refers to transfer of signals from one neuron to
another mediated electrically or chemically.
The neuromuscular endplate is the contact zone between the
axons of motor neurons and striated muscle fibres. The acetylcholine
containing vesicles of the axon terminals dock on the release sites of
the presynaptic membrane with high affinity. The muscle cell membrane at the
endplate is folded in junctional crypts. Nicotinic acetylcholine
receptors are concentrated at the openings of these crypts.
Plasticity refers to mechanical plasticity of smooth muscle tissue or to
an amplification produced by synapses, which transmit better when frequently
used.
Recruitment refers to the increase in force and contraction velocity of
a muscle by activation of more and more motor units.
Sarcomere is a contractile unit of a muscle fibril containing the halves
of two I-bands with the A-band in between (ie, the part of the fibril between
two neighbour Z-lines).
Synaptic transfer refers to the transmission of signals from one neuron
to another, and the site of contact between the two neurons is called the synapse.
Essentials
This paragraph deals with 1. Neuromuscular
junctions, 2. Synapses, 3. Skeletal muscles, 4. Smooth muscles and
5. Cardiac muscle tissue.
1.
Neuromuscular junctions
The neuromuscular endplate is the contact
zone between the axons of motor neurons and striated muscle fibres. Axon
terminals have vesicles containing acetylcholine (Fig. 2
-1). The
vesicles dock on the active zones or release sites of the
presynaptic membrane with high affinity. The muscle cell membrane at the
endplate is folded in junctional folds or crypts (Fig. 2-1). Nicotinic
acetylcholine receptors (Chapter 6) are
concentrated at the openings of these junctional crypts. The release sites are
located directly over the acetylcholine receptors (Fig. 2-1). The postsynaptic
membrane has acetylcholinesterase all over its surface.
The nicotinic acetylcholine receptor is related to a ligand
(acetylcholine)-gated ion channel found not only in the neuromuscular junction,
but also at all autonomic ganglia (Chapter 6) and in the central nervous system
(CNS). The receptor is fixed into the postjunctional membrane, whereas acetylcholinesterase is loosely attached to its surface. The receptor has five integral protein
subunits (2a , 1b , 1g , 1d ), surrounding a central ion channel pore that
is opened by the binding of 2 acetylcholine molecules to the 2 a - proteins (Fig. 2-1). Opening of the ion channel increases the conductance for
small cations (Na+ and K+) across the postjunctional
membrane, depolarising the membrane potential of the cell. These ion channels
are not voltage-gated (not dependent on changes in membrane potential), like
most cation channels in neurons, cardiac and skeletal muscle cell membranes.
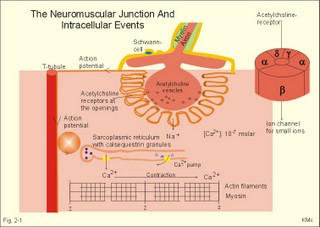
Fig. 2-1: The
neuromuscular junction and intracellular events. Acetylcholine = ACh. The
ACh-receptor to the right is magnified.
The acetylcholine-vesicles are probably already stored close to the release
zones, awaiting the release signal (Fig. 2-1). When the action potential
(AP) reaches the axon terminals, the axon membrane is depolarised, and
voltage-gated Ca2+-channels are transiently activated. This causes Ca2+ to flow down its concentration gradient from the outside into the axon terminal.
The influx of Ca2+ at the release zones causes the vesicles to fuse
with the axon membrane, and empty acetylcholine into the 50 nm wide cleft by exocytosis (Fig. 2-1).
After crossing the synaptic cleft by diffusion, acetylcholine binds to its receptor
protein on the muscle cell membrane. This binding complex opens the ion
channel and increases the conductance for small cations across the muscle cell
membrane. The influxes of Na+ depolarise the endplate temporarily, the transient depolarization is termed the endplate potential (EPP).
The EPP dies away when acetylcholine is hydrolysed to acetate and choline by the
enzyme, acetylcholinesterase. The EPP has a large safety margin, as a single
action potential in the motor axon will produce an EPP that always reaches
the threshold potential in the muscle fibre.
Rapid contraction of the muscle fibre is achieved by propagation of the
muscle action potential along the whole length of the muscle fibre membrane and
into the small, transverse tubules, which penetrate all the way through the
muscle fibre (T-tubules in Fig. 2-1).
The acetylcholine binding at the motor endplate increases endplate
conductance and generates an action potential (AP) in all directions from the
end plate (Fig. 2-1). The electrical excitation of the sarcolemma and the
transverse tubules (T-tubules) during the AP triggers – by an unknown
mechanism - the sarcoplasmic reticulum to release a pulse of Ca2+ (Fig. 2-1). The Ca2+-channels opens transiently in the vicinity of
each sarcomere (Fig. 2-1). The sarcoplasmic [Ca2+] increases from 10-7 to 10-6 M (which is the threshold). This Ca2+ diffuses to
the adjacent myofilaments, where they bind strongly to troponin C on the active
filament, and end the troponin-tropomyosin blockade. This enables cyclic
crossbridges to work as long as the high [Ca2+] is maintained,
whereby contraction occurs. A continually active Ca2+-pump returns Ca2+ to the sarcoplasmic reticulum, and another Ca2+-pump in the cell
membrane also reduces sarcoplasmic [ Ca2+] . Then the thin filament is off duty, because Ca2+ is
withdrawn from its troponin C, the troponin-tropomyosin-blockade is
re-established and relaxation ensues. The terminal cisternae of the
sarcoplasmic reticulum contain granules of calsequestrin, a protein that can
bind Ca2+ and reduce the concentration gradient (Fig.
2-1).
Neurons with motor function have the ability to
synthesise acetylcholine, because they contain choline-acetyltransferase.
This enzyme catalyses the production of acetylcholine from acetyl-CoA and
choline. Almost all cells produce acetyl-CoA and choline. Choline
is also actively taken up from the extracellular fluid via a mechanism
indirectly powered by the Na+-K+-pump. There is a 50%
reuptake of choline from the synaptic cleft; hence some choline must be
synthesized in the motor nerve.
The postjunctional membrane depolarizes spontaneously - resulting in
so-called miniature endplate potentials (MEP-potentials). A miniature
endplate potential is probably caused by the spontaneous release of a single
vesicle into the cleft. This is called quantal release.
An endplate potential is prolonged when cholinesterase-inhibitors are
present in the synaptic cleft. This is because these substances (eserine,
edrophonium, malathion, parathion etc.) inhibits the enzyme and thereby protects
acetylcholine from being hydrolysed by the enzyme. The life dangerous parathion
poisoning is described in chapter 6. Under normal conditions, the endplate
potential is terminated by the rapid hydrolysis of acetylcholine by
acetyl-cholinesterase.
Acetylcholine is a transmitter in the CNS, in all motor neurons,
in all preganglionic neurons of the autonomic nervous system and postganglionic
parasympathetic fibres, and in a few postganglionic sympathetic fibres. The
cholinergic receptor subtypes are shown in Table
6-2.
2.
Synapses
Chemical synapses prevail in humans,
but we also have electrical synapses in gap junctions.
A chemical synapse consists of a neuronal presynaptic terminal, a
synaptic cleft and a subsynaptic (or postsynaptic) membrane with associated
receptor proteins (Fig. 2-2). The chemical synapse is highly developed in the
CNS. It conducts the signal one way only, and has a characteristic synaptic
delay.
The presynaptic axon terminal typically broadens to form a bouton
terminaux (presynaptic terminal).
Fig. 2-2. A synapse
between a preganglionic and a postganglionic neuron.
- The action potential, originating in the CNS, depolarises the
axon membrane by selective influx of Na+ , which has a large
electrochemical gradient. Repolarization follows rapidly by selective K+-efflux
(Fig. 2-2).
- When the action potential reaches the presynaptic membrane, Ca2+ enters the terminal through voltage-gated Ca2+-channels.
- Vesicles containing transmitter, fuse with the presynaptic membrane and
release their contents of acetylcholine into the synaptic cleft (Ca2+-induced
exocytosis).
- Transmitter molecules (acetylcholine, ACh) diffuse across the synaptic cleft
and bind to specific receptors, which are located into the postsynaptic
membrane (Fig. 2-2). This ligand binding elicits a transient opening of pores, which are specifically permeable to small cations. The synaptic cleft of a
chemical synapse is about 30 nm.
- The ACh-receptor opens and allows influx of Na+, whereby the
membrane depolarizes and an action potential is generated which propagates
along the length of the postganglionic axon (Fig.
2-2). This is an appropriate
response of the postsynaptic cell to the received signal.
- The effect is rapidly terminated by the highly specific enzyme
acetylcholinesterase, which hydrolyses acetylcholine into two inactive
products (acetic acid and choline).
Influx of Na+ or efflux of K+ through the pores
of such receptors changes the postsynaptic membrane potential. If the
presynaptic action potential (AP) results in a postsynaptic depolarization, the
transient is called an Excitatory Post-Synaptic Potential (EPSP). If the
AP results in a postsynaptic hyperpolarization, the transient is called an Inhibitory
Post-Synaptic Potential (IPSP). Excitatory synapses often use glutamate as the transmitter. The pores are penetrated mainly by Na+, which
enters the cell, depolarizes the membrane, and produces an EPSP.
The axon hillock on the cell body has a high density of voltage-gated
Na+- and K+-channels. The axon hillock probably integrates
the many synaptic potentials, and from here the action potential is generated.
The dendrites have voltage-gated channels for K+ and for Ca2+.
Recent evidence suggests that dendrites also contain voltage-gated Na+-channels,
which are involved in electrogenesis (ie, movement of charge
across the membrane).
Each neuron in the CNS is in contact with up to 105 presynaptic
axon terminals. Synaptic inputs are integrated at the axon hillock by either spatial or temporal summation.
Spatial summation occurs when inputs from several axons arrive
simultaneously at the same postsynaptic cell. Their postsynaptic potentials are
additive. EPSPs summate and move the membrane potential closer to the threshold
level for firing. Conversely, EPSPs and IPSPs cancel each other out.
Temporal summation occurs when successive APs in a presynaptic neuron
follow in rapid succession, so that the postsynaptic responses overlap and
summate. Summation is possible because the synaptic potential lasts longer than
action potentials by a factor of 10-100 times.
Each individual synapse contains receptors, ion channels, and other key
molecules, which are sensitive to the neurotransmitters released at the site.
These specific protein molecules are involved in synaptic plasticity and
summation.
Electrical synapses. A gap junction is a transmembrane
pathway of low electrical resistance that connects the cytoplasm of adjacent
cells. A gap junction allows the membrane potential of the adjacent cells to be electrically
coupled. Gap junctions form electrical synapses, which differ
from chemical synapses in that transmission, is instantaneous.
An electrical synapse consists of several protein pores, which close in
response to increased intracellular [Ca2+] or [H+] in a
cell, thereby increasing their resistance. Open gap junctions exchange ions and
small molecules up to a molecular weight of 1000 Dalton.
Gap junctions are found in simple reflex pathways, where rapid transfer of
the electrical potential is essential, and between non-neural cells such as
epithelial and myocardial cells, smooth muscle cells and hepatocytes.
Neurotransmitters are divided into classical, rapidly acting
non-peptides (Table 7-1) and putative, slowly acting neuropeptides
(Table 7-2) - all
dealt with in Chapter 7.
Here is only described the function of GABA, neuropeptides and dopamine.
The major inhibitory transmitters are GABA (gamma-aminobutyric acid)
in the brain and glycine in the spinal cord. Binding of GABA to the
GABA-receptor opens the pore for Cl- influx, whereby the subsynaptic
cell membrane hyperpolarises (Fig. 2-3). The increase in Cl- conductance stabilises the membrane potential and decreases the efficacy of
excitatory transmission. The GABA-receptor pore is permeable to K+ besides Cl-. The GABA-receptor has a major inhibitory role in brain
function and is the binding site for barbiturates (used as hypnotics in
anaesthesia) and for benzodiazepines (used to relieve anxiety).
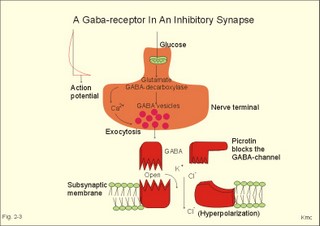
Fig. 2-3: A GABAA -receptor in an inhibitory synapse.
The GABAA-receptor shown here is related to sedation and mood, whereas the GABAB-receptor controls spasticity (Chapter 7). Picrotin blocks the GABA-channel.
Glutamate, aspartate and related acidic amino acids are the most
important excitatory transmitters in the brain and spinal cord.
Excitatory neurons possess excitatory amino acid (EAA) receptors. EAA
receptors are a family of receptors with at least four different ions channels:
The N-methyl-D-aspartate-receptor (NMDA), and three so-called non-NMDA
receptors - one of which is the glutamate receptor. The
NMDA-receptor operates with K+-efflux, while Na+ and Ca2+ enters the subsynaptic neuron. Mg2+ and many antiepileptic drugs
block the NMDA-receptor channel (Chapter 7). Opening of Na+- and Ca2+-channels,
which allow an increased influx of Na+ and Ca2+, cause the
membrane potential to approach the threshold level for excitation. Both a
reduced Cl--influx to the neuron and a reduced K+-efflux
move the membrane potential towards the threshold level and possible excitation.
The NMDA-receptor has a separate glycine site.
Neuropeptides (Table 7-2) have
slow excitatory or inhibitory transmitter actions. Peptides cannot be
synthesized locally in the axon terminals, because they do not have ribosomes.
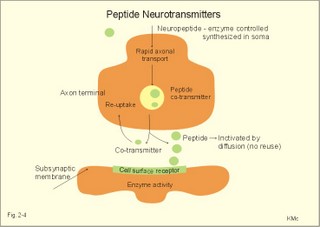
Fig. 2-4: Peptide neurotransmitters
Peptides are water soluble, and act as hormones by binding to specific
cell-surface receptors. Cell-surface receptors are a family of
guanosine triphosphate-binding proteins, so-called GTP-binding or G-proteins, which control and amplify the synthesis of second messengers. Cell-surface
receptors for neurohormones can function as transport protein and possess enzyme
activity (Fig. 2-4).
Neuropeptides are build by a sequence of amino acids. Neuropeptides are
synthesized in the cell bodies of the neurons and transported to the terminal
buttons by rapid axonal transport (Fig. 2-4). Some neuropeptides are released
together with a non-peptide co-transmitter (Table
7-2).
Some neuropeptides are produced when a large mother-peptide is cleaved
into several active neuropeptides. Neuropeptides are released from the nerve
terminal near the surface of its target cell, and diffuse to the receptors of
the target cell. Low concentrations of neuropeptides typically affect the
membrane potential by changing the conductance of the target cell to small ions.
The action of neuropeptides usually lasts longer than that of enzyme-inactivated
transmitters. Following prolonged synaptic transmission, neuropeptides are
deactivated by proteolysis.
Dopamine and other catecholamines derive from tyrosine via DOPA, which
stands for the precursor 3,4-dihydroxy-phenylalanine. Dopamine is the actively
accumulated into storage vesicles in the nerve endings together with
noradrenaline and ATP. Dopamine activates both presynaptic and subsynaptic D2-receptors
(Fig. 2-5).
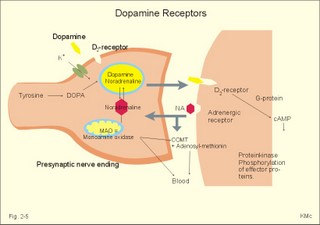
Fig. 2-5: Dopamine receptors and the interactions with noradrenaline (NA).
Noradrenaline can be oxidatively deaminated by monoamine oxidase (MAO)
located on the external membrane of mitochondria (Fig. 2-5). The enzyme COMT
(catechol-O-methyl transferase) can also methylate noradrenaline to
nor-metanephrine. MAO and COMT are important in metabolising circulating
catecholamines. Re-uptake of noradrenaline is the most important terminator of
its actions.
Activation of both D2-receptors opens K+-channels and
the increased outflux of K+ hyperpolarizes the membrane. Blockage of
the presynaptic D2-receptors in substantia nigra with antipsychotic
drugs reduces K+-outflux and increases dopamine production and
release.
Loss of dopamine-containing neurons in substantia nigra results in the
lack of dopamine at the D2-receptors of the striatal neurons. These
neurons degenerate in Parkinson's disease causing muscular rigidity and hand tremor (Chapter 4).
3.
Skeletal muscles
Skeletal or striated muscles are
attached to a skeleton. Striated muscles are called striated, because
they have a striking banding pattern. Microscopy with polarised light reveals dark (optically anisotropic) striations or A bands alternating with
light or optically isotropic striations or I bands. Running along the
axis of the muscle cell or muscle fibre is the myofibril bundles of filaments that are visible on electron micrographs. The A band
contains the thick filaments of myosin, and the I band contains thin
filaments of actin and tropomyosin (Fig. 2-6). The thin filaments are
anchored to a transverse structure termed the Z disc (Fig. 2-6). Each
contractile unit contains the halves of two I-bands with the A-band in between.
This unit is a sarcomere. Sarcomeres have a length of 2.3-2.5 mm
between the two Z discs at rest. The central A band is a relatively isotropic
substance - also termed the H-band - with an M-line of darkly
stained proteins that link the thick filaments into a fixed position.
Contraction takes place by sliding of the filaments.
The sliding of filaments against each other is called the sliding filament
hypothesis, and since contraction works by cycling of millions of
crossbridges, it is also called the theory of crossbridge cycling.
The thin filaments are 1-1.2 mm long
and consist of small globular proteins that form two helic pearl strings. The double
helix of actin is supported by a long, thin molecule of tropomyosin that is situated along the groove of the double strands of actin (Fig 2-6).
Each tropomyosin molecule interacts with 7 actin molecules on each side. Troponin is composed of 3 subunits: Troponin-C binds Ca2+, troponin-T
reacts with tropomyosin, and troponin-I inhibits the actin-myosin-interaction,
when Ca2+ is absent. Dystrophin is another normally occurring
cytoskeletal muscle protein.
The thick filaments are 1.6 mm long, and
consist of large myosin molecules. Myosin is a dimer of almost 500 kD. Each
monomer consists of one heavy chain and two light chains. The heavy chain consists of a helical tail and a globular head (Fig. 2-6). The light
chains are associated with the head of the heavy chain. Since myosin
is a dimer, the double-helix tail must end in two globular
heads (Fig. 2-6). The globular heads contain the ATPase activity and
the actin-binding site. The light chains control the rate of cross bridge
cycling.
Fig. 2-6:
Thick and thin filaments. The crossbridge cycle.
The crossbridge cycle theory states that there are multiple cycles of myosin-head attachment and detachment to actin during a muscle
contraction. When myosin binds to actin, an actinomyosin complex is formed -
with an extremely active ATPase. The interaction between actin and myosin and
the hydrolysis of ATP is the basic process that converts chemical energy into
mechanical energy.
Each crossbridge consists of two heads. At rest the crossbridge from myosin
is not attached to actin. The globular myosin heads are oriented perpendicular
to the filament axis (Fig. 2-6), and they
have a high standard affinity for actin.
- Stimulation of a muscle liberates Ca2+ in the sarcoplasma,
which removes the troponin-tropomyosin blockage of the actin, and actin can
react with the binding sites on the globular heads. The crossbridge is now
bound to the thin filaments (Fig. 2-6).
- The binding accelerates the release of ADP and Pi from the
actin-myosin complex, and the attached global heads change conformation by
45o with respect to the filament axis. The head of the
crossbridge drags the thick filament 10 nm along towards the Z-disc
or - at constant length - a proportional force is developed. Multiple
repetitions of this short sliding process is necessary to result in an
appreciable muscle shortening. In the absence of ATP, the crossbridge cycle
stops here and the binding is immobile (rigor link and rigor
mortis).
- The following stage is the binding of ATP to the myosin heads, which
weakens the binding to actin and disrupts the rigor link.
- Then ATP is partially hydrolysed on the myosin head, and the resulting
energy is stored in the perpendicular head, which has a renewed high
standard affinity for actin. If Ca2+ is present, a new
crossbridge cycle is initiated and may occur 100 times each s. With a cycle
movement of 10 nm this is 1000 nm per s for each half of the sarcomere.
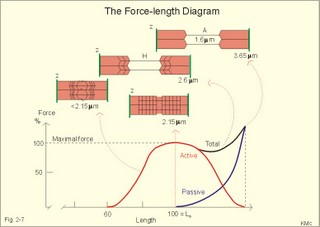
Fig. 2-7:
Force-length diagram
Force is required to stretch a relaxed muscle, because muscle tissue is
elastic, and the force increases with increasing muscle length (Fig. 2-7). The
passive blue curve reflects the properties of the elastic, connective tissue,
which becomes less compliant or stiffer with lengthening (Fig. 2-7).
A muscle contraction at constant length is termed isometric. Force is
measured in Newton (N), and one N is the force required to accelerate a
mass of one kg with an acceleration of one m s-2. In muscles,
the traditional expression for force is stress or tension in N per
cross-sectional area of the muscle (N m-2), which is actually
pressure (Pascal, Pa). Here, the ordinate is force expressed as a percentage of
the maximal force (Fig. 2-7).
1. The length at which maximum active contractile force is developed is
called Lo, corresponding to a sarcomere length of 2.15 m m (Fig. 2-7). Lo is the length of the muscle in the body when at
rest. At this length there is a maximum number of active crossbridges (Fig.
2-7). When an isolated muscle in an isometric force or stress-meter is stimulated, the active muscle force decreases with the decrease in overlap
between thin and thick filaments; at a sarcomere length of 3.65 m m the isometric force reaches zero (Fig. 2-7). The force is always proportional
to the number of cycling cross-bridges interacting with the thin filament.
2. Force also declines at muscle lengths less than Lo (Fig.
2-7). Thin filaments overlapping, and thick filaments colliding against Z-discs
cause this. The isometric force (stress) decreases as the sarcomere length is
reduced, as shown with the sarcomere length of less than 2.15 m m (Fig. 2-7).
3. When the active muscle length is stretched beyond any overlapping
between the thin and the thick filaments the muscle can only develop a force of
zero (see the sarcomere length of 3.65 m m with an
A-band of 1.6 m m in Fig. 2-7).
The lengths of the thick and thin filaments of human striated muscles are
similar (1.6 and 1.2 m m, respectively). They
generate maximal tension forces at Lo, corresponding to
a sarcomere length of 2.2 m m, namely 300 kN per m2 or kPa.
Muscle power or work-rate (Eq. 2-1)
is the product of muscle force (N) and shortening velocity (m s-1).
The maximal work rate of human muscles is reached at a contraction velocity of
2.5 m s-1. The maximal work-rate is thus (300 kPa *2.5 m s-1)
= 750 kW per square meter of cross sectional area.
Hill developed an equation for the shortening velocity of isotonic muscle
contractions (Eq. 2-2). The equation is illustrated in
Hills force-velocity diagram (Fig. 2-8).
The maximum force is developed at the initial length (Fig. 2-8 right: 18 g of
load). At 18 g there is no shortening – the length is unchanged. Stimulation
of the unloaded muscle results in maximum shortening velocity (100%). An
unloaded crossbridge can cycle at maximal rate, indicated by maximal shortening
velocity (Fig. 2-8 right).
The shortening velocity decreases rapidly as the afterload is increased
describing a hyperbola (Fig. 2-8 right). With increasing loads the latency is
increased and the shortening is reduced (4 and 9 g in Fig. 2-8
left). The
latency depends on the length of thepreceding isometric phase. The maximal
velocity of shortening is directly proportional to the myosin ATPase activity.
We increase the velocity of muscle shortening under a given load by the
recruitment of additional motor units.
The long human arm muscles shorten at a rate of 8 m per s. Muscles can bear a
load of 1.6 times the maximal force before the crossbridges are broken, but
under such extreme conditions the work-rate (power) of the muscle approach zero
(no shortening in Fig. 2-8 left). This is also the case when a person attempts
to lift a motor car - the speed of shortening is zero (isometric contraction).
On the other hand, the speed at which a pocket thief operates is probably
impressive, although the force is minimal.
Maximal work-rate occurs at a load of 1/3 of the maximal isometric force of
the muscle. Here the contractile system has optimal efficiency in converting
chemical energy into mechanical energy.
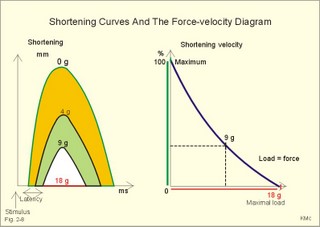
Fig. 2-8:
Hill's force-velocity diagrams (right) and related shortening curves (left).
A further rise in filament velocity seems to reduce the potential for
actin-myosin interaction. The crossbridge cycling rate falls as the load
on the crossbridges increases (Fig. 2-8 right).
In a muscle, the force of contraction is graded by increasing the
frequency of action potentials, and by recruiting more muscle cells.
Prolonged crossbridge contraction results in physiological tetanus. This
is a prolonged muscle contraction maintained by the prolonged Ca2+-influx
caused by repetitive stimulation.
Human skeletal muscles consist of three functional types of motor units. A
motor unit is a motor neuron with the muscle fibres it innervates. All muscle
fibres belonging to a motor unit are of the same type. The three types of muscle
fibres are characterised in Table 2-1.
Table 2-1. Structural, functional and histochemical
characteristics of twitch fibres. |
Classification |
Red (I) |
Red (IIA) |
White (IIB) |
|
Slow oxidative (SO) |
FOG |
FG |
|
Intermediate |
Red |
White |
|
Slow |
FR |
FF |
|
Slow-twitch |
Fast-twitch red |
Fast-twitch white |
Myoglobin |
High |
High |
Low |
Oxidative enzymes |
High |
Intermediate |
Low |
Glycolytic activity |
Low |
Low |
High |
Glycogen |
Low |
High |
Intermediate |
Mitochondria |
Intermediate |
High |
Low |
Mitochond.ATPase |
Intermediate |
High |
Low |
Sarcoplasmic retic. |
Intermediate |
Dense |
Dense |
Fibre diameter |
Small |
Intermediate |
Large |
Contractions |
Postural |
Endurance |
Powerful |
Shortening velocity |
Low (I) |
Intermed. (IIA) |
High (IIB) |
Recruitment |
First |
Second |
Last |
Most human skeletal muscles are a mixture of all three types of motor units,
although the proportions vary considerably.
Type I: The slow motor units contain slow-oxidative (SO) red slow-twitch fibres.They are adapted to continuous postural muscle activity. The fibres
have many mitochondria and a high content of myoglobin (red fibres). They depend
on aerobic metabolism and the glycogen content is high. Slow motor units have
weak but long lasting contractions (slow reaction to a signal or twitch). The
fibres are small and are first to be recruited. During light work these highly
excitable motor units activate red fibres suited for prolonged activity or
endurance activities. Endurance training increases the oxidative capacity of the
activated motor units, whereas strength training increases cellular hypertrophy.
Type IIA: Fast-twitch, fatigue-resistant (FR) motor units have
type IIA twitch fibres with a high or intermediate content of mitochondria,
myoglobin, and glycogen. These fibres also rely upon oxidative metabolism (fast
oxidative glycolytic = FOG) and have a high level of both oxidative and
glycolytic metabolism. The motor units provide contractions of intermediate
force and duration, and they resist fatigue. FOG fibres are of intermediate
size, and they are recruited before the white fibres. This is in accordance with
the size recruitment principle: Small or intermediate motor units are
easier to activate by excitatory postsynaptic potentials (EPSPs) than large
neurons.
Type IIB: Fast-twitch fatiguable (FF) motor units produce fast contractions
(fast-twitch), and fatigue easily, as the name implies. Their large white
fibres, with their dense sarcoplasmic reticuli, are adapted to activities
requiring large forces with rapid control of contraction and relaxation. The
fast-twitch white fibres (also called type IIB due to the highest shortening
velocity) have few mitochondria, small amounts of myoglobin (white fibres), and
depend on glycolysis (high anaerobic metabolism). They have only small amounts
of glycogen (fast glycolytic = FG). The FF motor neuron is large, the axon is
thick and it branches so greatly that the FF motor unit innervates more muscle
fibres. This is why FF motor units are capable of powerful contractions. The
cell body receives type Ia afferents. The FF units are recruited last and mainly
during maximal efforts such as sprinting. The production of ATP by glycolysis
matches the high rate of ATP consumption.
We have three major metabolic sources of ATP:
- Phosphocreatine, which is an immediate energy source used for intense
white fibre activity such as sprinting. Lohmann's creatine kinase catalyses
the efficient reforming of ATP from ADP by the conversion of a small
phosphocreatine pool to creatine. Following exercise the oxygen debt is repaid and the phosphocreatine pool is restored (Chapter
18).
- The glycogen stores of the muscle produce ATP rapidly but inefficiently by glycolysis, with lactate as the end product.
- Glucose, free fatty acids, triglycerides and amino acids in plasma are
substrates for oxidative phosphorylation. This is a most efficient pathway
and the slowest source of energy due to the many steps in the process (Chapter
20).
4.
Smooth muscles
The same molecules as in striated muscle essentially
cause contraction in smooth muscle, but the intracellular organisation and the
dynamic characteristics are entirely different (Table 2-2).
Table 2-2: Characteristics of skeletal, cardiac and
smooth muscle cells. |
|
Skeletal |
Cardiac |
Smooth muscle |
Diameter (m m) |
Up to 100 |
10 |
Up to 5 |
Length (m m) |
200 000 |
50 |
Up to 200 |
T-tubules |
Yes |
Yes |
No -Simple caveoli |
Regular sarcomers |
Distinct |
Distinct |
No -Look smooth |
Regular Z-discs |
Yes |
Yes |
No- but dense bodies |
Regular myofibrils |
Yes |
Yes |
Irregular myofibrils |
Troponin |
Yes |
Yes |
No |
Sarcoplasmic reticulum |
Yes |
Yes |
Simple reticulum |
Gap junctions |
No |
Yes |
Yes (single-unit) |
Extracellular Ca2+ |
No |
Yes |
Yes |
Refractory period |
Short |
Long (300ms) |
Long |
Latency (ms) |
10 |
10 |
200 |
Twitch (ms) |
10-100 |
300 |
3000 |
Resting membrane pot.( mV) |
-80 |
-90 |
-50 |
Force |
High |
High |
Low maintained for days |
Energy cost |
300-fold |
High |
Low |
Disorders |
Atrophy |
Cardiac |
Asthma, hypertension |
Smooth muscles are called so because they lack the distinct sarcomeric
bands of striated muscles. Smooth muscle cells are spindle-shaped and
line the hollow organs and the vascular system; the smooth muscle cells are
extremely small (Table 2-2). Smooth muscle cells contain a few thick
myosin-filaments, and many thin actin-filaments attached to dense bodies by a-actin (helical sarcomers). The cells are without regular sarcomers, Z disc's, myofibrils and T-tubules. Smooth muscle cells lack
troponin. Dense bodies are analogous to Z disc's, and some dense areas are
attached to the cell membrane. Smooth muscle cells do not contain a typical
sarcoplasmic reticulum, which can store and release Ca2+. Instead
some fibres possess an analogous simple reticular system located near the caveoli of the cell membrane. Caveoli are small invaginations of the
membrane, similar to the T-tubules of striated muscles. The more extensive the
reticular system is in the smooth muscle fibre, the higher is its shortening
velocity due to release of Ca2+ mediated by IP3. Smooth
muscle cells maintain large forces almost continually at extremely low energy
costs.
The same tension or tone is maintained for days in smooth muscle organs
(intestine, urinary bladder, gall bladder) and can be obtained in striated
muscle at high energy cost (up to 300 times the smooth muscle rate of ATP
consumption).
Smooth muscle cells are extremely sensitive to extracellular [Ca2+].
During an action potential the inward flux of ions is not Na+, but
Ca2+ through slow Ca2+-channels. They open mainly in
response to a ligand binding, but we have also voltage-dependent Ca2+-channels.
The force-length relation is qualitative similar to that of striated muscles,
so the sliding-filament mechanism is probably analogous (Fig.
2-6).
The smooth muscle mechanism is special, because stimulation results in a
maintained isometric force with strongly reduced velocities. Smooth muscle
contractions are extremely slow. Ca2+ probably regulates the number
of active crossbridges in smooth muscle slowly and indirectly.
Smooth muscle cells contain some mitochondria, and they show a
slow contraction pattern superimposed on the lasting tonus. Smooth muscle
contractions typically last for 3 s, in contrast to striated muscle with total
contraction periods of 10-100 ms. Since the energy demand in smooth muscle is
extremely low, it is balanced by the oxidative ATP synthesis. Smooth
muscle cells do not have an oxygen debt as striated muscles do, although they
produce large amounts of lactate. This is probably because the
ATP-synthesising glycolytic mechanism is located in the cell membrane and is
linked to the ATP-utilising Na+-K+-pump. Smooth muscle
contains far fewer myosin filaments than striated muscle. The myosin crossbridge
heads of smooth muscle contain an isoenzyme with much less ATPase activity than
that of striated muscle. Ca2+-entry through the cell membrane is much
slower than internal release of Ca2+.
A contracting smooth muscle fibre releases Ca2+ from two pools.
The large extracellular fluid pool is essential. In the fibre that
possesses a sarcoplasmic reticulum similar to the sarcoplasmic reticulum
of striated muscle, there is a fast intracellular pool. The smooth muscle cell
membrane contains a 3Na+-2K+-pump, a delayed K+-channel, a ligand-activated and a voltage-dependent Ca2+-channel, a
sarcolemmal Ca2+-pump, and a Na+- Ca2+-exchanger
(Fig. 2-9).
- A stimulatory ligand is bound to membrane receptors for G-proteins
and for ligand-gated Ca2+-channels (Fig. 2-9). The major Ca2+-influx
takes place through the ligand-gated (noradrenaline) and the voltage-gated
Ca2+-channels. The Ca2+-influx depolarizes their
membrane, whereby Ca2+ further permeates the membranes. The
depolarization by ligand binding thus indirectly opens the voltage-gated
channels.
- When a stimulus acts on reticular receptors via a G-protein it activates
phospholipase C. Phospholipase C hydrolyses phosphatidyl inositol
diphosphate (PIP2) into IP3 and diacyl-glycerol,
DAG (Fig. 2-9).
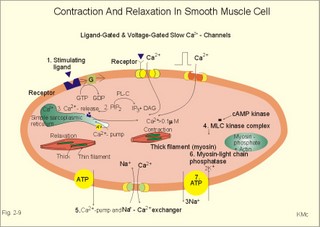
Fig. 2-9: Contraction
and relaxation in smooth muscle cells. The ligand is acetylcholine in visceral
cells and noradrenaline, ATP and peptide hormones in vascular smooth muscle
cells.
- IP3 is bound to a receptor on the simple sarcoplasmic reticulum
and this second messenger binding elicits a controlled release of Ca2+ from the reticulum. Hereby, the sarcoplasmic [Ca2+] rapidly
increases above the threshold for contraction (0.1 m M).
- The crossbridge cycling is regulated by a myosin light chain
kinase (MLC kinase) dependent upon both Ca2+ and
calmodulin. The phosphorylation of myosin to myosin-phosphate is drastically
accentuated by the binding of 4 Ca2+-calmodulin to MLC kinase
forming a complex. The phosphorylated light chain myosin reacts with actin
in the thin filaments and contracts. The rate of sliding and of
ATP-splitting is up to 1000-fold slower than in striated muscles.
- Ca2+ is actively pumped out of the cell by an ATP-demanding Ca2+-pump
and through a Na+- Ca2+-exchanger (antiport). The
antiport uses the energy of the Na+-gradient for influx. Reuptake
into the poorly developed sarcoplasmic reticulum and the mitochondria is
slow compared to cardiac and skeletal muscle tissue.
- Below the Ca2+-threshold the myosin light chains are
dephosphorylated by myosin light chain phosphatase and the contractile
structures relax.
- The Na+-K+-gradient across the cell membrane is
maintained by the Na+-K+-pump (Fig.
2-9).
When the high intracellular [Ca2+] during an action potential is
lowered again towards the resting level, the cell relaxes. This is accomplished
by stimulation of the sarcolemmal Ca2+-pump, and by blockade
of both Ca2+-input and Ca2+-release.
Metarterioles and precapillary sphincters without nerve fibres can still
respond to the needs of the tissue by the action of local tissue vasodilatators.
The following factors cause smooth muscle relaxation, and therefore
vasodilatation: Adenosine, NO, lack of oxygen, excess CO2, increased
[H+], increased [K+], diminished [Ca2+], and
increased [lactate].
Endothelial-derived relaxing factor (EDRF) is recently shown to be nitric
oxide (NO). Activation of endothelial cells produces NO from arginine, and
NO diffuses into the smooth muscle cells. NO stimulates directly the enzyme guanylate-cyclase,
and by that intracellular [cGMP] elevates.
Circulating acetylcholine contracts the arterial smooth muscles
when bound to cholinergic receptors.
Smooth muscle cells grow (hypertrophies) as a response to the needs of the
body, and they also retain the capacity to divide.
During hypertension the lamina media of the arterioles hypertrophies
which increases the total peripheral vascular resistance in the systemic
circulation. These topics are further developed in Chapter
9.
During pregnancy the (single-unit, see below) smooth muscles of the
myometrium are quiescent and contain few gap junctions under the
influence of progesterone. At term the myometrium grows and the number of gap
junctions increases, due to the high oestrogen concentration. Now the
myometrium is well prepared for the co-ordinated contractions during parturition (see Chapter 29).
Smooth muscle changes length without marked changes in tension. Initially,
there is a high tension developed upon stretching; then the tension falls as the
myosin and actin filaments are reorganised by slowly sliding against each other.
A sudden expansion of the venous system with blood results in a sharp rise in
pressure followed by a fall in pressure over minutes. The smooth muscle fibres
in the walls of the venous system are highly compliant, because they have
accepted a large blood volume without much rise in pressure (delayed
compliance).
Smooth muscle cells are frequently involved targets
in diseases such as hypertension, stroke,asthma, and many gastrointestinal
diseases.Smooth muscle cells can be divided into multi-unit smooth muscle and
single-unit smooth muscle.
Fig. 2-10: Contraction of multi-unit smooth muscle cells (vascular). A single contraction
is elicited by an electrical stimulus and later acetylcholine elicits tetanus.
Contraction of multi-unit smooth muscle is controlled by extrinsic innervation
or by hormones. Mechanical contact junctions between the cells are not found.
- In multi-unit smooth muscle tissues each cell operates entirely
independent of other cells and the cell does not communicate with other muscle
cells through gap junctions. The discrete cells are separated by a thin
basement membrane and often innervated by a single neuron, and their main
control is through nerve signals. Thousands of smooth muscle cells belonging
to the multi-unit type join by the common innervation in a functional
syncytium. Multi-unit smooth muscle is found in the eye (the
ciliary muscle and sphincters as the iris muscle of the eye), in large
arteries, in the vas deferens, and in the piloerector muscles that cause
erection of the hairs. These muscle cells are normally quiescent, insensitive
to stretch and they are activated only through their autonomic nerves. Each
muscle is composed of multiple motor units, hence the name: multi-unit
smooth muscles. The nerve fibre branches on a bundle of smooth muscle
fibres, and form junctions with varicosities filled with transmitters.
These junctions are analogous to the neuromuscular junctions of striated
muscles. The neurotransmitters are acetylcholine and noradrenaline. Multi-unit
smooth muscles have developed a contact junction with shorter latency
than the slowly operating diffuse junctions mainly found in the
single-unit type.
- Single-unit smooth muscle cells are arranged in bundles such as the
arrangement in a viscera eg. intestine, uterus and ureter (Fig. 2-11). These
smooth muscle cells commun-icate through hundreds of gap junctions,
separating the cell membranes by only 2-3 nm, and from pacemaker tissue of
variable location, action potentials are generated initiating a contraction of
the muscle. In this respect single-unit cells resemble the cardiac muscle.
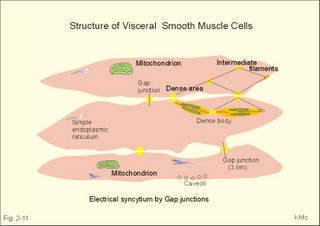
Fig. 2-11:
Single-unit smooth muscle cells resemble cardiac muscle. Activity propagates
from cell to cell through gap junctions forming an electrical syncytium. The
dense bodies and dense areas contain alpha-actin.
Action potentials generated in one cell can activate adjacent cells by ionic
currents spreading rapidly over the whole organ and securing a co-ordinated
contraction as though the tissue were a single unit or a syncytium.
These cells are characterized by their spontaneous motility and by their
sensitivity to stretch. The spontaneous activity is usually modified by the
autonomic nervous system. Visceral smooth muscle undergoing peristalsis,
generates propagating action potentials from cell to cell.
Other cell-to-cell contacts are desmosome's and intermediary
junctions subserving structural contact. These intermediary junctions
transfer mechanical force from one smooth muscle cell to another on the plasma
membrane, causing the single-unit smooth muscle cell to function like a stretch
transducer.
5.
Cardiac muscle tissue
Myocardial cells are built of regular sarcomers just
like the skeletal muscles, and they are contracting fast. Myocardial cells form
an electrical syncytium in the same way as the single-unit smooth muscle cells.
The characteristics of myocardial, skeletal and smooth muscle cells are
presented in Table 2-2. Myocardial cells are mononuclear and the myoglobin,
enzymatic and mitochondrial content are large just as the red fibres of skeletal
muscles. The metabolism of myocardial cells is similar to that of red skeletal
fibres, both being designed for endurance rather than speed and strength. The
oxygen supply to the heart muscle must be maintained, if it is to synthesise ATP
at a sufficient rate. Myocardial cells deprived of oxygen for 30-s cease to
contract.
Myocardial cells most resembles smooth muscle in its auto-rhythmicity and
syncytial function. Pacemaker cells in the sinus node determine the normal
cardiac frequency, because they send out spontaneous action potentials along the
conduction system of the heart with a higher frequency than any other cells in
the heart. Vagal stimulation releases acetylcholine at the pacemaker cells.
Acetylcholine increases the K+-permeability, whereby K+ leaves the cell and hyperpolarizes the cell membrane. This is why the pacemaker
(cardiac) frequency is reduced by vagal nerve stimulation. Sympathetic
stimulation or adrenaline reduces the K+-permeability, so the
depolarization is shortened, and the pacemaker frequency increased.
The prolonged action potential characteristic for myocardial cells is
initiated by an abrupt Na+-influx (phase 0) through fast Na+-channels just as in the striated muscles. The AP plateau is due to a slow Na+-Ca2+-channel,
which deliver Ca2+ for the contraction activation. The action
potential releases Ca2+ from the sarcoplasmic reticulum to the
sarcoplasma. The effect is distributed by the cardiac T-tubule system.
Cardiac contraction by crossbridge cycling depends on the presence of
extracellular Ca2+ just as in smooth muscle tissue. Therefore, use of
Ca2+-antagonists reduces the contractile force of the heart, whereas
drugs, which increase Ca2+-permeability across the membrane, improve
the contraction. In the heart, Ca2+-influx tends to prolong the
depolarization just as in smooth muscle cells. The cardiac glycoside, digoxin,
selectively binds to and inhibits the sarcolemmal 3Na+-2K+-pump,
which leads to an increase in intracellular [ Na+] . Although the Na+-efflux is inhibited, the redundancy of Na+ affects the Na+-Ca2+-exchanger (3 Na+ out
for one Ca2+ into the cell), leading to an increase in cellular [ Ca2+] and in the force of contraction.
This is the mechanism of the increase in contractile force by digitalis
glycosides.
Pathophysiology
This paragraph deals with 1. Disorders
of the neuromuscular junctions (myasthenia gravis), 2. Skeletal
muscle disorders (dystrophia, dystonia, muscle injuries), 3. Smooth
muscle disorders (asthma, hypertension etc) and 4. Myocardial
disorders (coronary artery disease, arrhythmias, and chronic heart disease).
1.
Disorder of the neuromuscular junction (Myasthenia gravis)
This serious disease is acquired, but the cause is
unknown. The development of this autoimmune disorder may be
related to other diseases. Rheumatoid arthritis treated with D-penicillamine has
resulted in myasthenia gravis. More than 50% of the myasthenia patients have thymic
hyperplasia and some patients have a real thymoma.
Many of these patients have an increased blood concentration of antibodies
against their own acetylcholine receptor protein. There is a decreased
density of receptor proteins on the postjunctional membrane. This was shown
by the use of radioliganded toxins from poisonous snakes (which bind
irreversibly to the acetylcholine receptor protein).
The patients are tired and the muscles are extremely weak. This is
particularly so for the proximal limb muscles, the extraocular muscles and the
neck muscles, whereby the patient has difficulties in lifting the head.
Mastication and swallowing is a difficult process.
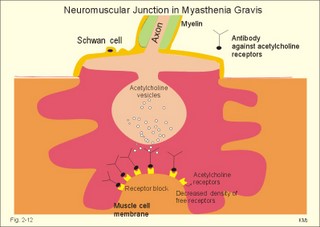
Fig. 2-12:
Neuromuscular junction with antibodies and decreased density of
acetylcholine-receptors in a patient with myasthenia gravis.
As just mentioned the blood of most patients with myasthenia gravis contains autoantibodies against acetylcholine (ACh) receptor proteins on the cell surfaces of
the motor end plates etc. The autoantibody competes for the ACh receptor and
inhibits synaptic transmission, so muscular contraction is greatly inhibited.
Deposition of immune complexes eventually destroys the ACh-receptor protein.
Intravenous injection of an anticholinesterase improves the muscle
strength immediately, but the beneficial effect is gone within 3 min.
Thymectomy improves the condition and the prognosis also in the group of
patients without thymoma.
Oral anticholinesterase (such as pyridostigmine) has beneficial effect
over 2-4 hours. They inhibit the enzyme acetylcholine-esterase, and thereby
prolong the effect of naturally occurring acetylcholine on the receptors. In
severe cases this treatment is inefficient, and immune-suppressants such as
corticosteroids are sometimes favourable.
2.
Skeletal muscle disorders
Muscular dystrophy is an inherited disorder of
skeletal muscles. Duchenne muscular dystrophy is an X-linked recessive
muscle disorder characterized by the absence of dystrophin in the
striated muscles and in the myocardium. The locus is localised to the Xp21
region of the X chromosome. Dystrophin is a normally occurring cytoskeletal
muscle protein. The patient is a boy, who has to climb up his legs in order to
reach the erect posture. Typically, there is proximal weakness with compensatory pseudohypertrophy of the calves. There is no cure and the patient dies
from myocardial damage.
Dystonias are prolonged muscle contractions leading to muscular
spasms. There is a simultaneous action of opposing agonist and antagonist groups
that produce abnormal postures. Dystonia is painful and particularly resistant
to treatment.
Dystonia musculorum deformans begins in childhood with generalized spasms
that affect gait and posture. In most cases the cause is a genetic defect.
Spasmodic torticollis causes the head to turn (torticollis) or change
posture. Patients with a trigger zone on the jaw benefit from acupressure here.
Muscle injuries are dealt with in Chapter
18.
3.
Smooth muscle disorders
The most important disorders are asthma (Chapter
14) and systemic hypertension (Chapter
12). Smooth muscles are also
involved in a disorder of swallowing (achalasia), where the myenteric
plexus and the lower oesophageal sphincter fail to respond with receptive
relaxation, and the food accumulates in the oesophagus. Other disorders of
the gastro-intestinal smooth muscles are also treated there.
4.
Disorders of the myocardium
Coronary artery disorders (smooth muscles and
myocardial disease) and congestive heart disease are treated in Chapter
10, and
cardiac arrhythmias in Chapter 11.
Only direct therapeutic uses of the systems developed till now are described
here.
Nitro-glycerine, nitroprusside and similar drugs relax smooth muscles by
transfer of NO from endothelial cells. NO increases intracellular [cGMP]
(Fig.11-1), which is the basis for the beneficial effect of the drugs on cardiac
cramps. These second messengers activate protein kinases that phosphorylate
effector proteins such as Ca2+-pumps and K+-channels. Such
vasodilatators stimulate the sarcoplasmic Ca2+-pump, inhibit
Ca2+-influx and stimulate K+-efflux through the delayed K+-channel
(reduces the excitability). Hereby, the high intracellular [Ca2+]
during an action potential is lowered towards the resting level (10-7 mM), and the smooth muscle cell relaxes producing vasodilatation.
Equations
Muscle power (or work rate) equals the product of muscle force and
shortening velocity
Eq. 2-1: Power (W) = Force (N) * Velocity (m s-1).
- Hill’s equation.
The force-velocity curve is shown in Fig.
2-7. The
curve fits Hill’s equation:
Eq. 2-2: Initial shortening velocity (v) = (Po -
P)*b/(P + a)
where P is the force or load acting on the muscle, Po is the maximal
isometric force or load, a is a constant with the dimensions of a force,
and b is a constant with the dimensions of velocity.
Self-assessment
Multiple
Choice Questions
I. Each of the following five statements have False/True options:
A. Motor neurons synthesise acetylcholine unrelated to their content
of choline-acetyltransferase.
B. There is a high density on the subsynaptic membrane of specific
acetylcholine receptors.
C. The receptor protein for acetylcholine contains a voltage-gated
channel for cations.
D. Binding of acetylcholine elicits a transient opening of ionophores,
which are specifically permeable to small ions.
E. Parkinson's disease is possibly caused by loss of dopamine
containing neurons in the substantia nigra.
II. Each of the following five statements have False/True options:
- Nitro-glycerine, nitroprusside and similar drugs contract smooth muscles
by transfer of nitric oxide from endothelial cells.
- All myasthenia patients have a thymoma.
- During hypertension the lamina media of the arterioles hypertrophies which
increases the total peripheral vascular resistance in the systemic
circulation.
- The nicotinic acetylcholine receptor is related to an acetylcholine-gated
ion channel found not only in the neuromuscular junction, but also at all
autonomic ganglia and in the central nervous system.
- When the high intracellular [Ca2+] during an action potential
is lowered again towards the resting level, the cell contracts. This is
accomplished by stimulation of the sarcolemmal Ca2+-pump, and by
blockade of both Ca2+-input and Ca2+-release.
Try to solve the problems before looking up the answers.
Highlights
- Recruitment is the increase in force and contraction velocity of a muscle
by activation of more and more motor units.
- Synaptic transfer refers to the transmission of signals from one neuron to
another, and the site of contact between the two neurons is called the
synapse.
- A chemical synapse consists of a neuronal presynaptic terminal, a synaptic
cleft and a subsynaptic membrane with associated receptor proteins. The
chemical synapse is highly developed in the CNS. It conducts the signal one
way only, and has a characteristic synaptic delay.
- A gap junction or electrical synapse is a pathway of low electrical
resistance that connects cytoplasm of adjacent cells. A junction couples
adjacent cells electrically and thus allows synaptic transmission without
delay.
- Neurons with motor function have the ability to synthetize acetylcholine,
because they contain choline-acetyltransferase.
- GABA (gamma-aminobutyric acid) in the brain and glycine in the spinal cord
are inhibitory neurotransmitters. Binding of GABA to the GABA-receptor opens
the pore for Cl- influx, whereby the subsynaptic cell membrane
hyperpolarizes. The GABA-receptor has a major inhibitory role in brain
function and is the binding site for barbiturates (used in anaesthesia) and
for benzodiazepines (used towards anxiety).
- Glutamate, aspartate and related acidic amino acids are the most important
excitatory transmitters in the brain and spinal cord. Excitatory neurons
possess excitatory amino acid (EAA) receptors. These EAA-mediated synapses
predominate in the CNS.
- Each neuron in the CNS is in contact with up to 105 presynaptic
axon terminals. Synaptic inputs are integrated by either spatial or temporal
summation.
- Neuropeptides are built by a sequence of amino acids. Neuropeptides are
synthesized in the cell bodies of the neurons and transported to the
terminal buttons by rapid axonal transport.
- Loss of dopamine-containing neurons in substantia nigra results in lack of
dopamine at the D2-receptors of the striatal neurons. These
neurons degenerate in Parkinson´s disease causing muscular rigidity and
hand tremor.
- Blockade of the presynaptic D2-receptors in substantia nigra
with antipsychotic drugs reduces K+-outflux and increases
dopamine production and release.
- The crossbridge cycle theory states that there are multiple cycles of
myosin-head attachment and detachment to actin during a muscle contraction.
When myosin binds to actin, an actomyosin complex is formed - with an
extremely active ATPase.
- Muscle power or work-rate is the product of muscle force (afterload in N)
and shortening velocity (m s-1). The maximal work rate of human
muscles is reached at a contraction velocity of 2.5 m s-1. The
maximal work-rate is thus (300 kPa *2.5 m s-1) = 750 kW per
square meter of cross sectional area.
- Tetanus is a prolonged muscle contraction maintained by the prolonged Ca2+-influx
caused by a high stimulation frequency.
- Smooth muscle cells are frequently involved targets in diseases such as
hypertension, stroke, asthma, and many gastrointestinal diseases.
- Smooth muscle cells maintain large forces almost continually at extremely
low energy costs. The same tension or tone is maintained for days in smooth
muscle organs (intestine, urinary bladder, and gall bladder).
- Myocardial cells form an electrical syncytium in the same way as the
smooth muscle cells do.
- Myocardial cells deprived of oxygen for 30-s cease to contract.
- The most important smooth muscle disorders are asthma and hypertension.
- The most important myocardial disorders are coronary artery disease,
arrhythmias, and chronic heart disease.
- Myasthenia gravis is a disorder of neuromuscular contraction. The patients
frequently have an increased blood concentration of antibodies against their
own acetylcholine receptor protein and thymic hyperplasia.
- Duchenne muscular dystrophy is an X-linked recessive muscle disorder
characterized by the absence of dystrophin in the striated muscles and in
the myocardium.
Further
Reading
Kupfermann, I. "Functional studies of cotransmission." Physiol.
Rev. 71: 683, 1991.
Pollack, G.H. "Muscles and molecules: Uncovering the principles of
biological motion." Seattle, Washington, 1990. Ebner & Sons.
Alberts, B. et al. "Molecular biology of the cell." 4th Ed.,
2002, Garland Publishing, Inc., New York & London.
Return to top
Return to Content
|