Study Objectives:
· To define pH, pK, PaCO2, acids, bases, buffers, buffer bases (titratable
base), actual bicarbonate and non-carbonic buffers, the extended extracellular
fluid volume (ECV extended with red cells), and the base excess of the
extended ECV.
· To describe the daily acid-base balance, titratable acidity and the net excretion of acid
in the urine, the base excess of the blood and extended ECV, and the buffers
of the intracellular fluid volume.
· To calculate the third variable of the Henderson-Hasselbalch equation from two measured
variables.
· To draw the acid-base charts (ie, a double logarithmic plot of the
Henderson-Hasselbalch equation).
· To explain the acid-base chart, metabolic processes involved in the regulation of pH,
tubular H+-secretion, and renal bicarbonate reabsorption. To
describe intracellular buffers and pH, transport of H+ across the
cell membrane. To describe four acute disorders of the acid-base status (primary respiratory and metabolic acidosis and alkalosis) and their
chronic counterparts with therapeutic suggestions.
· To use the acid-base variables in problem solving and diagnosis of acid-base
disorders.
Principles
· The
mass action equation. Blood plasma is an open system where gasses are in
equilibrium both with the alveolar air in the lungs and with the extracellular
fluid volume (ECV). ECV and all red cells work as a buffer unit called
extended ECV. Bicarbonate -carbonic acid is the strongest buffer and
haemoglobin is the strongest non-carbonic buffer. The pH of arterial blood
plasma is a function of PaCO2 and of the concentration of
titratable buffer bases in the extended ECV (Table 17-1). The concentration of
titratable base (Base Excess, BE) is measurable, and BE remains zero during
acute changes of PaCO2.
· The
disorders of the acid-base balance are described by the following two
interrelated chemical reactions:
CO2 + H2O Û*
H2CO3 Û H+ + HCO3- and
[ Acid form] « H+ + [ Non-carbonic buffers-]
Non-carbonic buffers - or
non-bicarbonate buffers - refer to all other relevant buffers than the
carbonic acid-bicarbonate buffer system. The enzyme carbo-anhydrase is shown
with an asterisk: *.
Definitions
· Acid (HB) is defined as a compound that can release a hydrogen ion or proton
(H+), whereas a base (B-)
can bind H+.
· Acidosis (acidaemia) is defined as a disorder with accumulation of acids in the
extended ECV. The pH measured in the arterial blood is less than 7.35.
· Alkalosis (alkalaemia) is defined as a condition with accumulation of bases in the
extended ECV. The pH of the arterial blood is larger than 7.45.
· Actual
bicarbonate concentration is
the concentration calculated from PaCO2 and pH measurements in the arterial blood sample. The value can be calculated
with Eq. 17-6 or read from an acid-base chart with a bicarbonate axis (slope
-1 in Fig. 17-3).
· The
acid-base buffer capacity of
a system is defined as the amount of strong acid or base added to one litre
(l) of the system (ie, mmol per l or mM) in order to change the pH one unit.
· A buffer is a corresponding acid-base pair, which acts as a pH-stabiliser.
· Buffer
base (BB) refers to the total
concentration of all carbonic buffer anions plus the non-carbonic anions.
· Carbonic
acid refers to carbon
dioxide.
· Carbonic
buffers refer to the carbonic
acid-bicarbonate buffer system.
· Glomerular
filtration is due to a
hydrostatic/colloid osmotic pressure gradient - the Starling forces
· The
acid-base buffer capacity of the extended
ECV is normally 61 mM per pH unit as an average (Table
17-1).
· Non-carbonic
buffers (non-bicarbonate buffers) refer
to all other buffers than the carbonic acid-bicarbonate buffer system in the
extended ECV (Table 17-1).
· The
Base Excess (BE) of the
extracellular fluid (extended ECV) is calculated as the concentration
difference of buffer bases in the actual sample and in the same sample
following titration with strong acid or base and equilibration to standard
conditions (pH 7.4, PaCO2 5.3 kPa
or 40 mmHg). The difference is
given in mmol of strong acid or base, which must be added to 1 l of the
sample. The base excess remains normal (zero) in acute respiratory acid-base
disorders
· Extended
extracellular fluid volume (extended
ECV) is the extracellular fluid volume (ECV) plus the erythrocyte volume.
A useful model of the extended ECV is
an arterial blood sample diluted three folds with its own plasma (ie, 2+1).
Extended ECV behave as a functional buffer unit. As a model extended ECV is
considered to be all red cells distributed evenly in the extracellular fluid
volume. The extended ECV of a
healthy standard person covers approximately 20% of body weight. The principal buffers of
the extended ECV in a healthy person
are shown below (Table 17-1). Extended ECV serves as an early distribution
volume in acid-base disorders.
· Intracellular
fluid volume (ICV minus red
cells) comprises approximately 40% of the body volume. The principal buffers of ICV are proteins, phosphate and bicarbonate. The transport across cell
membranes take hours to equilibrate and the intracellular buffer effect is
involved in the delayed distribution. The intracellular buffer effect is
larger in disorders with negative base excess (acidosis with pH approaching pK
for the best buffers) than with positive base excess. OH- has a molecular weight 17 times larger than that of H+,
so H+ diffuses faster through the cell membrane, since the
diffusion rate depends on the hydrated ionic radius
· pH is defined as the negative logarithm to the proton concentration. In
this Chapter the pH is measured with glass electrodes in the arterial blood
sample, and used in the extended ECV
· pK is equal to pH, when the concentration of acid and of base is the same.
· Partial
pressure of carbon dioxide in
the arterial blood sample (PaCO2)
is measured with glass electrodes, and PaCO2 refers to the extended ECV in this Chapter.
· Metabolic
acidosis is acidosis with negative Base Excess. - Negative Base Excess means that the numeric
difference between the measured buffer base (BB) and the normal buffer base
(NBB) is negative.
· Metabolic
alkalosis is alkalosis with positive Base Excess. - Positive Base Excess means that the numeric
difference between the measured buffer base (BB) and the normal buffer base
(NBB) is positive
· Metabolic
acid-base disorders are
caused by changes of the titratable base of the extended ECV (Base Excess is
positive or negative).
· Nephron - the functional renal unit. Each nephron consists of a glomerulus, a
proximal convoluted tubule, a Henle loop and a distal tubule ending in a
collecting duct with several other nephrons.
· Respiratory
acid-base disorders are
caused by alterations of PaCO2,
without any change of the amount of titratable base in the extended ECV (BE is
zero).
· Respiratory
acidosis is an acidosis with
a PaCO2 above 6.4 kPa or 48 mmHg at rest
· Respiratory
alkalosis is an alkalosis
with a PaCO2 below
4.4 kPa or 33 mmHg at rest.
· Titratable
phosphate acidity in the
daily urine is the amount of base (mmol) needed to titrate an acidic urine
(phosphoric acid) back to the pH of plasma and glomerular filtrate (pH = 7.4
and PCO2 of 5.3 kPa).
· Tubular
reabsorption is the movement
of water and solute from the tubular lumen to the tubule cells and to the
peritubular capillary network
· Tubular
secretion represents the net
addition of solute to the tubular lumen.
Essentials
This paragraph deals with 1. Proton concentration & pH, 2. Buffer capacity and the
bicarbonate buffer , 3. Buffer
capacities, 4. From the H-H-equation to the
acid-base chart, 5. Extended ECV & Base excess, 6. Metabolic acid-base production, 7. Renal acid-base control, 8. Intracellular buffers.
1.
Proton concentration and pH
Normally,
the [H+] of arterial plasma of
humans at rest is maintained by the lungs and kidneys within the range of 40 ± 5 nM, corresponding to a pH of 7.36-7.44. The [H+] of the arterial
plasma is also maintained during strenuous conditions and diseases: 16-160 nM
or pH 6.8-7.8 (Fig. 17-1). Proton
concentrations in nM differ by a factor 10-6 from the normal
standard-[Na+],
which is around 140 mM.
A
pH of 6.8-6.9 is not sustainable for long, and the patient is dying in a state
of coma. In contrast, a healthy athlete can survive a pH of 6.85 following
supramaximal exercise with headache as the only consequence.
A
pH approaching 7.8 implies dissociation of all buffer proteins in order to
produce H+ (Eq. 17-7), and negative albumin charges capture Ca2+,
so the extracellular Ca2+ falls and the patient dies in tetanic
cramps including laryngeal spasms (Fig. 17-1). Tetanic cramps are spontaneous prolonged or continuous
muscular contractions (for explanation see later)
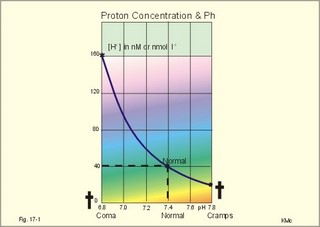
Fig. 17-1: Relationship between pH
and the proton concentration
2. Buffer capacity and
the bicarbonate buffer
The acid-base buffer capacity of a system is defined as the amount (mmol) of strong acid or base added
to one litre (l) of the system (ie, mmol per l or mM) in order to change the
pH one unit
The
importance of the CO2/bicarbonate buffer is easily realised when
comparing the addition of one mmol of strong acid to one litre of a closed and
an open system with 24 mM bicarbonate each (pH 7.4; PCO2 5.3 kPa).
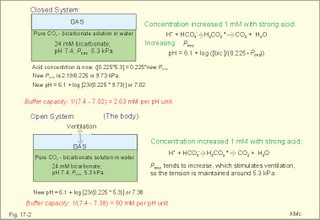
Fig. 17-2: The CO2/bicarbonate buffer
working in a closed and an open system.
In the closed system, the
base concentration is reduced by 1 mM to 23, and the acid concentration is
increased by one mM, because the reaction is shifted towards formation of CO2 causing a high PCO2 (Fig. 17-2). Accordingly, the acid concentration is now: ([0.225 × 5.3] +
1) = 0.225 × newPCO2.
Thus the newPCO2 is 2.19/0.225 or 9.73 kPa. The pH of the closed system is changed to 7.02 (Fig. 17-2). The buffer capacity is
1/(7.4 - 7.02) = 2.6 mM per pH unit, which is negligible.
In an open system such as
the body, the ventilation simply eliminates excess carbon dioxide and the PCO2 is kept constant: 5.3 kPa or 40 mmHg. The chemoreceptors are bathed in
extracellular fluid. Any rise in the PCO2 of the extracellular fluid is sensed by the chemoreceptors and releases a
proportionate rise in ventilation. Thus, the new
pH is 7.38 (Fig. 17-2). The buffer
capacity is at least: 1/(7.4 - 7.38) = 50
mM per pH unit, which is an essential capacity (see Table
17-1)
3. Buffer capacities
The buffer capacity of a system is already defined as the amount of strong acid or base added
to one litre (l) of the system in order to change the pH one unit
The most important buffer
system for the regulation of [H+] in extended ECV is the carbonic
acid (CO2)/bicarbonate system (82%), although its pK is 6.1 and not
so close to ideal as for the primary/secondary phosphate system (Table 17-1).
The CO2/bicarbonate buffer is
distributed in the extended ECV (up to 15 l of CO2 is bound as bicarbonate). Its buffer
capacity is high, since PaCO2 is rapidly maintained normal by respiration, and the kidneys control bicarbonate excretion.
Table
17-1: Principal buffer concentrations and their contribution to the
buffer capacity of the extended ECV in a healthy person. |
|
Net-anion
Concentration (mM, mean) |
Buffer
capacity (mM per pH unit) |
Bicarbonate |
24
(67%) |
50
(82%) at constant PaCO2 |
Non-carbonic
buffers |
12
(33%) |
11
(18%) |
Haemoglobin |
7 |
9 |
Plasma Protein |
4 |
2 |
Phosphate |
1 |
0.4 |
Total |
36
(100%) |
61 (100%) |
Of
the non-carbonic buffer bases (18%), the haemoglobin has the dominating buffer value with 9 mM per pH unit in
the extended ECV, leaving only about
2 mM per pH unit for proteins (essentially albumin) and phosphate (Table 17-1).
4. From The H-H-equation
to the Acid-Base Chart
The
three variables in the final Henderson-Hasselbalch equation (pHa, PaCO2,
and actual bicarbonate in Eq. 17-6) are easy to handle. Rearrangement of Eq. 17-6, with constant bicarbonate concentration,
shows the following:
log PaCO2 = - pH + k or y = -x + k,
where k stands for three constants (6.1 + log [constant bicarbonate] - log 0.03).
Thus,
y is a linear function of x, and the slope of the line is -1. This slope is
called an iso-bicarbonate line, and the slope reflects the buffer capacity
(rise in carbonic acid per pH unit) of the bicarbonate buffer in water.
Siggaard-Andersen
has developed a double logarithmic plot, where factor 10 has the same distance (decade equidistant). The pH is
chosen as the abscissa (pH = 6.8 - 7.8) and log PaCO2 is the ordinate (10-100 mmHg in Fig.17-3).
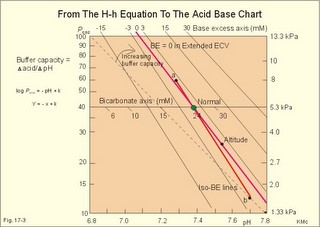
Fig. 17-3: The pH-log PaCO2 plot (decade equidistant).
All
points on each iso-bicarbonate line in the plot with a slope of -1 have the
same bicarbonate concentration. An example is the iso-bicarbonate
line relating pH and log PaCO2 in a pure bicarbonate solution in water (15 mM) - shown by a stippled line with the slope -1 in Fig.
17-3. Here the
bicarbonate concentration is constant (included in k)
namely 15 mM in all points. Such iso-bicarbonate lines are pure mathematics.
When two variables are given, the third variable is readable from the plot (or
easy to calculate according to the equation).
Originally,
the equilibration line for a blood sample was determined by equilibration with
high and low PCO2 as
shown by the points a and b, crossing a bicarbonate axis in mM running through PaCO2 40
mmHg (= 5.3 kPa in Fig 17-3). The actual
bicarbonate concentration is readable at this bicarbonate axis. As an
example, let us assume that the point b,
characterises the actual pH and PaCO2 measured. An iso-bicarbonate line is drawn with the slope -1 (use a piece of
folded paper) and the actual bicarbonate for the point b is read on the bicarbonate
axis to be 15 mM. This is
simply a fast geometrical calculation
Adding the non-carbonic buffers of
whole blood (thick red line between a and b in Fig. 17-3) or of extended
ECV (purple line) to a pure bicarbonate solution in water, naturally increases
the buffer capacity. These lines are therefore steeper than the
iso-bicarbonate lines (-1). The most important of the non-carbonic buffers is
haemoglobin in the red cells (Table 17-1).
The
actual bicarbonate concentration is dependent of variations in PaCO2.
When PaCO2 increases,
carbonic acid is buffered by non-carbonic buffers causing a rise in actual
bicarbonate (see the two interrelated reactions in Principles). The elevated actual bicarbonate is interpretable as a metabolic
alkalosis, also when a respiratory
acidosis is the real cause. The interpretation is a serious error
Accordingly,
we have to look for a better index to separate metabolic from respiratory
acid-base disturbances
The
steepest equilibration line illustrates the buffer capacity for whole-blood
buffers (Fig. 17-3 a to b). The slope of an equilibration
line for all the buffers in the
extended ECV is slightly smaller than that of whole-blood, because the
whole-blood buffers are diluted in the large extended ECV (Fig. 17-3, purple
line).
Base Excess (BE) of the extracellular fluid (extended ECV) is calculated as the
concentration difference of buffer bases in the actual sample and in the same sample following equilibration to standard conditions
(pH 7.4, PaCO2 5.3 kPa or 40 mmHg and well oxygenated). A healthy
person with these actual values for pH and PaCO2 must have a BE of zero.
As PCO2 rises due to hypoventilation the [bicarbonate] rises, but BE of the extended ECV remains zero. The slope of the BE- zero line depends primarily on the concentration of haemoglobin, less on the plasma
protein concentration, and minimally on the effect of inorganic phosphate (Table
17-1). The position of the BE-line depends
on the BE: adding strong acid shifts the line to the left, and adding base
shifts the line to the right. The series of almost parallel iso-BE-lines, with negative and positive values around the BE-zero
line, has been determined experimentally. Each iso-BE-line is an equilibration line for a standard person in a given metabolic
condition.
The
iso-BE-line zero depicts the
condition with normal concentration and buffer capacity of buffer bases, and
the other almost parallel lines represent abnormal conditions with either
positive or negative base excess. With pH, and PCO2 measured, it is easy to read the precalculated base
excess from the base excess axis at
the top of the acid-base chart (Fig. 17-13).
5. Extended ECV &
Base excess
The
extended ECV is the extracellular fluid volume plus the red cells. An
abstraction is to think of all red
cells distributed in the total extracellular fluid volume. A useful model of the extended
ECV is an arterial blood sample
diluted three folds with its own plasma (2+1).
The BE is independent of PaCO2,
since any change in PaCO2 implies opposite molar changes of the bicarbonate and the non-carbonic buffer
concentrations. Hereby, there is no change in BE. Thus, the base excess is
constant (normally equal to zero) during acute changes in PaCO2 by hyper- or hypoventilation.
The buffer capacity of
the carbon dioxide- bicarbonate buffer is high, since respiration and bicarbonate excretion by the kidneys rapidly
maintain PaCO2.
The base excess is a key variable in
diagnosing metabolic disorders. The
base excess tells the whole story, whereas the bicarbonate buffer only tells
part of the story (Table 17-1) - and sometimes in error. This is what makes Base
Excess the diagnostic choice.
BE alterations from zero are therefore used to diagnose metabolic,
as opposed to respiratory acid-base
disorders.
Ole Siggaard-Andersen also introduced the term 'concentration of titratable hydrogen ion' as an alternative to base excess. The 'Titratable Hydrogen Ion concentration Difference' can be shortened to THID, which is equal to base excess. The 2 terms simply represent the same concentration difference from normal in mM, but with opposite signs. Base excess is discharged, since the essential variable is not a base and in reality is not always an excess. Hence, from now on THID is used instead of base excess. THID in normal subjects is equal to zero, positive in primary, metabolic acidosis, and negative in primary, metabolic alkalosis.
6. Metabolic acid-base
production
The daily metabolic
production of carbon dioxide is up to 24 mol. The CO2 is a
potential acid as H2CO3, and because the lungs eliminate
it, it is called a volatile acid.
The hepatic production of
non-volatile acid depends upon its daily amino acid load from intestinal
absorption including amino acids from the daily degradation of intestinal
mucosal cells. Persons on a normal mixed diet produce up to 100
mmol fixed or non-volatile acids daily mainly from amino acids (plus
organic acids as uric acid, phosphoric acid, and minimal amounts of HCl and
sulphuric acid)
Persons
on a mixed diet absorb approximately 50 mmol organic bases daily (RCOO- in Table 17-2). A maximum of 20 mmol of these organic anions is excreted in a
24-hour urine, because at least 30 mmol react with H+ produced by
the liver. As stated above, the hepatic oxidation of sulphydryl groups in
amino acids and hydrolysis of phosphate esters from lipids produces 100 mmol H+ daily, of which 60-70 mmol is excreted in a 24 hour urine (Table 17-2).
Table
17-2: Absorption, renal excretion and metabolic
acid-base production (mmol daily) |
Input |
Non-volatile acids |
Non-volatile bases |
Gut absorption: |
0 |
50 (RCOO-) |
Metabolic production: |
Up to 100 |
Self-elimination |
Total: |
100 |
50 |
Output (renal excretion): |
NH4+/ NH3 -buffer |
30 (400 in acidosis) |
NH3 in
basic urine |
Phosphate (mainly from diet) |
30 (60 in acidosis) |
|
RCOO- |
|
20 |
Bicarbonate |
|
1-3
(100 in alkalosis) |
Total: |
60 |
21 |
As
stated above, the remaining 30 mmol of metabolic H+ is eliminated
daily by oxidation of 30 mmol organic bases from the gut (RCOO-).
About
30 mmol NH4+ is excreted in the daily urine, but the
excretion is controlled during acid-base disorders. During acidosis, the high
[H+] stimulates hepatic glutamate- and renal NH4+ -production, and the renal NH4+ -excretion increases
towards 400 mmol daily. During alkalosis the urea production accounts for the
nitrogen elimination and only negligible amounts of NH4+ is
excreted
Diets rich in vegetables
and fruits generate non-volatile bases (salts of organic acids such as oxalate
and citrate), which must be metabolised or excreted im the kidneys. In the
body only amino acids are metabolised to bases (ammonia). Urinary excretion of
the base form of ammonia takes place only in extremely basic urine.
Normally, there is no
non-volatile acid-base production from carbohydrates or lipids.
7. Renal acid-base
control
The
kidneys prevent the loss of enormous amounts of bicarbonate in the urine,
produce the most important urinary buffer (NH3/NH4+ -buffer), and excrete the titratable phosphate acidity. - The free H+ is not a major issue, since even acid urine with a pH =5 only eliminate 1/100
mmol per l
The details of paragraph 7.1 to
7.3 are best understood following a careful study of Chapter 25.
7.1 Bicarbonate
reabsorption
Normally, the daily
filtration flux of bicarbonate amounts to 4500 mmol (ie, filtration of 180 l
of plasma with a mean concentration of 25 mM). Most of the filtered
bicarbonate flux is reabsorbed already in the proximal tubules, where the
luminal membrane contains a Na+-H+-antiporter (Fig. 17-4). The bicarbonate reabsorption is accomplished by means of H+-secretion. Most of
the H+ secreted in the proximal tubules is derived from the Na+-H+-exchange through the antiporter. When the tubular fluid reaches the collecting
ducts an important H+-secretion is mediated by a proton-K+-ATPase in the intercalated cells (see below).
Any change in the
filtered bicarbonate flux is matched by a similar change in proximal
bicarbonate reabsorption (ie, glomerulo-tubular
balance). A change in Na+ - homeostasis alters the bicarbonate
reabsorption secondarily.
The Na+-K+-pump in the basolateral membrane provides the energy for the secretion
of H+ into the tubular fluid. This secretion serves to reabsorb the
filtered bicarbonate, which is thus not excreted in the urine. The daily
tubular secretion of H+ is enormous, because we excrete 70 mmol of
non-volatile acid and also have to match almost all of the total filtration
flux of bicarbonate. At the brush border of the proximal tubule cell, carboanhydrase (CA) catalyses the reaction, so CO2 is formed and can enter the
cell easily by diffusion (Fig. 17-4).
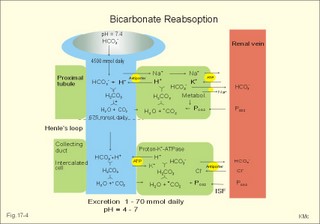
Fig. 17-4: Reabsorption of bicarbonate in the proximal and distal parts
of the nephron.
Also
within the cell CA facilitates the production of (H+ + HCO3-).
For each bicarbonate produced in the cell from the CO2 of the
tubular fluid, one bicarbonate ion diffuses to the interstitial phase and the
renal venous blood back to the body (Fig. 17-4).
The
cells of the thick ascending limb of the Henle loop also reabsorb bicarbonate
by the same mechanism as in the proximal tubule
The
small residue of bicarbonate enters the distal tubules, where it is reabsorbed
almost totally through a special mechanism independent of Na+ . In
the intercalated cells of the
collecting ducts, the reabsorption is dependent on a proton-K+-ATPase (Fig. 17-4). The bicarbonate ion crosses
the basolateral cell membrane in exchange of chloride through a chloride-bicarbonate antiporter.
- This special mechanism is most likely ineffective in distal
renal tubular acidosis.
Acidosis,
which involves the intracellular space and stimulates production of proton-K+-ATP-ases,
also favours H+ -secretion. Hereby, bicarbonate reabsorption is
stimulated, whereas alkalosis inhibits bicarbonate reabsorption by the
opposite mechanisms.
Aldosterone
stimulates the proton-K+-ATPases of the intercalated cells and the Na+-reabsorption/
K+ -secretion of the principal cells. Both effects favour H+ -secretion and thus bicarbonate reabsorption.
7.2 The NH3 /NH4+ -buffer
As
stated above, the most important urinary buffer is NH3/NH4+.
This is because the synthesis of NH3/NH4+ in
the tubule cells is controlled by the acid-base status of the body. Acidosis stimulates NH4+-production
from renal glutamate, whereas alkalosis stimulates hepatic urea production
from hepatic glutamate
In
a normally dieting adult person the amino acid load is from 90 g of protein
(16% nitrogen) daily. The (90* 0.16)= 14.4 g nitrogen (1 mol) corresponds to
1000 mmol of NH3 /NH4+. According to the
equation:
2
NH4+ + 2 HCO3-® CO(NH2)2 +
CO2 + 3 H2O
One
mol of nitrogen daily produces 500 mmol urea, which is equal to the typical
urinary urea excretion. The daily urea filtration flux is 900 mmol (5 mM * 180
l of plasma each day). The degree of reabsorption of the water-soluble urea
depends upon the tubular flow rate
Under
normal conditions only a small amount of nitrogen is used to produce hepatic
glutamate, which is an excellent atoxic ammonia store that can transfer
ammonia to the proximal tubules of the kidneys in cases of acidosis.
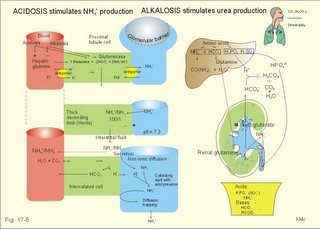
Fig. 17-5: Glutamate metabolism
and renal ammonia production. The renal handling of ammonia and the excretion
by diffusion trapping is shown.
In
the proximal tubules of the kidneys, renal glutamate produces NH4+ and a-ketoglutarate.
One molecule of NH4+ is produced by deamination of one
glutamine molecule by the enzyme, glutaminase, and a second by oxidative
deamination of glutamic acid forming a -ketoglutarate that is metabolised. The NH4+ in the
proximal tubule cells is in equilibrium with minimal amounts of NH3 at the relatively low pH. The NH4+-secretion into the
tubular fluid makes use of the Na+-H+-antiporter,
where NH4+ substitutes H+. The NH4+ passes with the tubular fluid to the thick ascending limb of the Henle loop,
where a major portion is reabsorbed and accumulated in the interstitial fluid
(Fig. 17-5).
Secretion
of NH4+ in the collecting ducts involves a special
mechanism. The NH3 is lipid soluble and easily passes any membrane,
so it reaches the tubular fluid of the collecting ducts and form NH4+ at the low pH (Fig. 17-5). The charged molecule cannot pass the membrane and
it is trapped in the tubular fluid and eliminated in the urine. This diffusion
trapping of charged molecules such as NH4+ is called non ionic diffusion - a general elimination principle for many
charged metabolites and drugs. Excretion of NH4+ reduces
the excretion of other positive ions.
The a -ketoglutarate is metabolised into bicarbonate. Bicarbonate of the
extracellular fluid reacts with H+ from hepatic phosphoric and
sulphuric acid to form carbon dioxide and water. The H2PO4- (and a minimal amount of SO42-) is excreted in the
urine. On a mixed diet the production and excretion of non-volatile acids and
bases results in a net excretion of acids equal to the daily net production of
non-volatile acids.
With
a urine pH of 6.5, organic acids such as lactic acid, b-hydroxybutyric acid, pyruvic acid etc., are present in the base form
(RCOO- of Fig. 17-6). Most of the phosphoric acid is H2PO4-,
and almost all ammonia is in the NH4+ form.
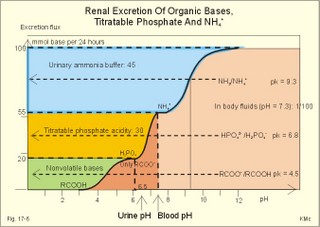
Fig. 17-6: Excretion flux for
organic bases (RCOO-), titratable acid (H2PO4-),
and NH4+ in normal daily urine.
This
is not so in an alkaline urine. At a urinary pH of 8, there is 5% NH3 of the total, just as in ileum and colon, where the pH is also 8.
The
high pK (=9.3) of NH3/NH4+ has the
consequence that in gastric juice with a pH of 1, the (pH-pK)- difference is
-8.3, so virtually all ammonia must be NH4+. Even in
body fluids with a pH of 7.3 the NH3/NH4+ ratio is 1/100.
7.3 The titratable
phosphate activity
The
dominating buffer system in the urine is secondary/primary phosphate. This is
because of its urinary concentration and of its pK (6.8) being close to
urinary pH (6.5).
A
healthy person has a renal filtration flux of phosphate of 180 mmol daily (1
mM*180 litres of plasma filtered daily). Phosphate is a threshold substance,
which is reabsorbed, in the proximal tubules, where parathyroid hormone (PTH)
inhibits phosphate reabsorption (Fig. 17-7). With 30 mmol left in the tubular
fluid, the secondary/primary phosphate-ratio is 24/6 mmol as calculated in Fig.
17-7. Secretion of H+ during the passage of the fluid through the
renal tubules converts HPO42- to the acid form, H2PO4-. Thus, in the final urine, the base/acid-ratio is 10/20 (Fig. 17-7). Titratable phosphate acidity in the daily urine is the amount of base (mmol) needed to titrate an
acidic daily urine back to the pH of plasma and glomerular filtrate (pH 7.4).
Weak acids are not titrated, because they are minimally dissociated at pH 6.5
to 7.4. Normally,
the titratable phosphate acidity is 30 mmol in a 24 hour urine. In our example
above, the distal H+-secretion has titrated 14 mmol of HPO42- to H2PO4- (Fig. 17-7). Acidosis increases the urinary titratable
phosphate acidity (towards 50 mmol daily) in order to get rid of the acid.
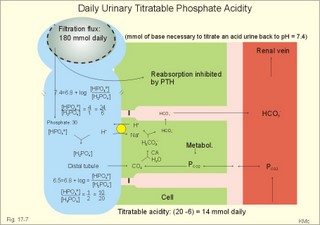
Fig. 17-7: Titratable phosphate acidity produced in the distal tubules.
The
small amount of bicarbonate in the daily urine (zero to 3 mmol) hardly affects
the measured titratable phosphate acidity, and the ammonia buffer is not
titrated in acid urine
8. Intracellular buffers
The
importance of the buffer capacity of the extended
ECV, and that of the intracellular fluid is comparable in the majority of
acute conditions, with extended ECV as the initial distribution volume and the
intracellular fluid participating importantly after hours. This is because the
alteration of the cellular transport processes takes time.
The
[H+] of the intracellular fluid volume (ICV) is higher than that of the extended
ECV. The intracellular pH is precisely controlled in cells with different
functions and needs, and the range of values is 7.0 -7.4 (Fig.
17-8).
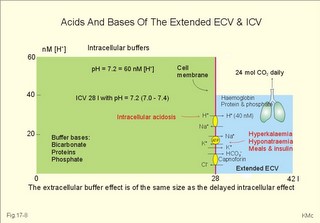
Fig. 17-8: The proton
concentrations and buffer bases of the extended ECV and of the ICV.
The
buffer bases within the cells are proteins, phosphate and bicarbonate. The
precise intracellular control is necessary for the pH-sensitive cellular
processes with pH-optima for the enzyme systems. The active transport of H+ out of the cell is a coupled Na+/H+ -exchange. The energy for this
exchange is delivered by the Na+-K+-pump, which
maintains the Na+ -gradient across the cell membrane (Fig. 17-8).
Carbohydrate- and K+-containing meals, insulin, hyperkalaemia,
adrenaline and aldosterone stimulate the Na+-K+-pump.
A
bicarbonate-transport protein (capnoforin in the red cell and in many other
cell membranes) transfers bicarbonate to the extended ECV by bicarbonate/chloride
exchange (Fig. 17-8).
In
disorders with extracellular accumulation of carbon dioxide, CO2 diffuses rapidly into the cells. This causes a shift towards the right in Eq.
17-4. The intracellular buffers buffer the H+. Bicarbonate leaves
the cells both directly via capnoforin and via other membrane channels (Fig.
17-8), whereby intracellular bicarbonate falls. Intracellular acid
accumulation accompanied by hyperkalaemia may develop, if not compensated by
the lungs. The K+-output follows the bicarbonate exit
Non-volatile acid is also
buffered intracellularly during metabolic acidosis by movement of H+ into
the cell, where it reacts with proteins, phosphate and bicarbonate. During
metabolic alkalosis movement of H+ out of the cells in exchange of
Na+ (Na+-influx in Fig. 17-8) also buffers non-volatile
base.
Pathophysiology
Humans can suffer from 4
acid-base disorders: 1. Respiratory
acidosis, 2 Respiratory
alkalosis, 3 Metabolic acidosis and 4 Metabolic alkalosis.
Acidosis (acidaemia) is defined as a disorder with pH in the arterial blood (pHa)
less than 7.35, and alkalosis (alkalaemia
or baseosis) is defined as a condition with a pHa larger than 7.45.
Each
of these two disorders has respiratory and metabolic forms.
Respiratory
acid-base disorders are caused by primary changes of PCO2, and
compensated by altered renal excretion of acid in a matter of days.
Metabolic acid-base
disorders are caused by primary changes in BE, and compensated partially by
the lungs in a matter of hours. The final correction of metabolic disorders is
always renal and takes several days.
Let
us now return to the four primary acute disorders and their four chronic forms:
1. Respiratory Acidosis
is
caused by hypoventilation (or
breathing of CO2 containing air). Hypoventilation is associated
with an impaired ability to
eliminate CO2, whereby PaCO2 increases and the accumulated CO2 reduces the arterial pH.
For
each mol of bicarbonate produced, one mol of non-carbonic buffer base is
eliminated, which means that Base Excess (BE) is unchanged zero (Fig. 17-9). The slope of the THID -zero line depicts the
buffer-base capacity of the extended ECV.
Any
primary respiratory disorder is compensated renally over days. This is because the high intracellular [H+]
increases the glutaminase synthesis and activity, the renal ammonia
production, the urinary H+-excretion (mainly NH4+ but also H2PO4-), with a virtually complete
reabsorption of filtered bicarbonate. Hereby, BE becomes positive during
compensation (arrow in Fig. 17-9). Recall BE is now THID but with inverse signs, but for didactic purposes we use BE.
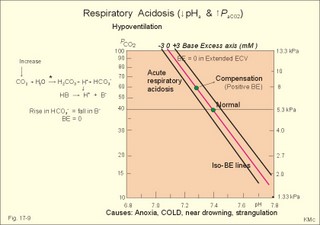
Fig. 17-9: Acute respiratory
acidosis with a base excess of zero, and its compensation.
In
severe cases of chronic CO2 accumulation, artificial ventilation is
necessary. This is often the case in the terminal phase of chronic obstructive
lung disease (ie, chronic bronchitis and emphysema). Other causes of
respiratory acidosis are asthma, pulmonary cancer or tuberculosis, polio, drug
overdose, anaesthesia, strangulation, near drowning and myasthenia gravis.
2. Respiratory Alkalosis is caused by hyperventilation.
The
hyperventilation is disproportionately high compared to the CO2 production, whereby the PaCO2 falls and the pH increases (Fig.
17-10). When the
alveolar ventilation is doubled, the PaCO2 is halved. This is a typical reaction to high altitude. As the PIO2 falls with increasing altitude, the PaO2 eventually falls below 55 mmHg, which stimulates the chemoreceptors to
hyperventilation (CO2 -wash-out).
Other
typical cases are the anxious patient during an attack of asthma or the
hysterical hyperventilation in neurotic patients. These patients often
experience tetanic cramps (see
below)
Hyperventilation
before underwater swimming eliminates the CO2 stimulus and shifts
the oxyhaemoglobin dissociation curve towards the left. Hereby, oxygen is
bound firmly to haemoglobin. When the PaO2 falls below 30 mmHg (4 kPa), blackout and grey-out occurs. Loss of
consciousness below water is often fatal.
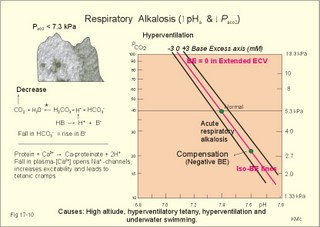
Fig. 17-10: Acute respiratory alkalosis and its compensation.
For each mol of
bicarbonate eliminated, one mol non-carbonic buffer base is formed, which
means that THID is maintained at zero (Fig. 17-10). As long as no non-carbonic acid or base is added to the extracellular fluid volume, the
extracellular base excess remains unchanged (zero)
Acute
respiratory alkalosis is compensated by increased renal excretion of bicarbonate, which
is the result of decreased tubular H+ -secretion. This is because
the low PaCO2 reduces the
tubular H+-secretion, and the alkalosis inhibits formation and
secretion of NH4+ . The renal
mechanisms affect the production and activity of cellular enzymes and
hormones, so it takes days to become effective.
After
a few days the renal compensation of the respiratory alkalosis is complete,
and the pH is normal. This is called totally
compensated respiratory alkalosis. - In cases of asthma-anxiety or
hysteria with hyperventilation tetany, simple rebreathing from a bag cures the
disorder within minutes.
Dissociation
of protein molecules occurs in all types of alkalosis in order to liberate H+.
The dissociation leads to tetany:
Protein + Ca2+ ® Ca-proteinate + 2 H+. The
equilibrium dislocates towards the right in alkalosis. The falling
extracellular [Ca2+] activates Na+-Ca2+-pumps and opens Na+-channels
in the cell membranes of neurons, muscle cells and the myocardial syncytium.
The Na+-influx reduces the membrane potential and increases the
excitability of the tissues, which causes tetanic cramps (almost continuous
muscular contractions).
3. Metabolic Acidosis
is
caused by accumulation of strong acids in the extended ECV. Metabolic acidosis
is diagnosed by negative base
excess, because both types of
buffer bases are reduced. In Fig. 17-11 both types of buffers and the total
concentration of buffer bases of the extended ECV are clearly reduced and the
BE is -15 mM.
The
iso-base excess-line (-15 mM) is steeper than the base excess-zero line, whereas the iso-base excess-line (+15 mM) is less steep than the BE-zero-line, due to the relative low
pK-values of essential buffers.
Strong
acids accumulate, because of excess production or impaired H+ -excretion.
Hunger
(hunger diabetes), diabetic
ketoacidosis, lactic acid accumulation or high protein intake with increased
production of hydrochloric and sulphuric acid, cause excess
production.
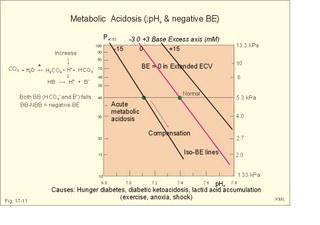
Fig. 17-11: Acute metabolic acidosis: The base excess is reduced. The
compensation is shown with an arrow.
Impaired renal H+ excretion is related to
increased loss of bicarbonate in the urine (due to renal failure). Diarrhoea
causes acidosis by loss of bicarbonate with
the faeces. Any loss of bicarbonate in the urine or faeces is equivalent to an
addition of H+ to the extracellular fluid (Fig. 17-11).
Lactic acidosis is caused by increased lactic acid production during exercise, shock, anoxia
or following cardiac arrest. Another type of lactic
acidosis is caused by decreased hepatic lactate metabolism - often
drug-induced.
Renal tubular acidosis is a damage of tubular cells caused by drugs or immunological reactions - or
it may be inherited. The impaired H+ secretion reduces the tubular
bicarbonate reabsorption. Kidney disease with destruction of a large number of
nephrons reduces the tubular capacity to excrete H+ and NH4+ in the urine. The chloride-bicarbonate antiporter of the intercalated cells of
the collecting ducts is probably ineffective (see above).
The
patient with metabolic acidosis suffers from dyspnoea (deep and frequent Kussmaull
respiration). This hyperventilation is a respiratory compensation, which develops over hours as a reduction
in PaCO2 (Fig. 17-11). This compensation is caused by the chemoreceptors, which
are surrounded by the extended ECV and stimulated by its hydrogen ion
concentration to react with hyperventilation.
Acidosis
shifts the oxygen dissociation curve to the right (ie, the Bohr effect),
increasing the delivery of oxygen to the tissues. Acidosis stimulates K+ -loss from the cellular pool, because of K+ -efflux from the cells
(Fig. 17-4). Chronic acidosis,
however, inhibits 2,3-DPG production, which tends to shift the oxygen
dissociation curve back towards the left
When
renal function is normal, K+ -loss from the cellular pool may lead
to K+ -deficiency. When renal K+ -secretion is impaired,
the cellular K+ -efflux may lead to hyperkalaemia.
Oxygen enriched air is administered in cases of lactic
acidosis with poor tissue bloodflow. Insulin must be given in diabetic
ketoacidosis.
A
patient with metabolic acidosis and a base excess of -15 to -25 mM may have to be
treated with bicarbonate infusion. The primary strategy is to eliminate the lack of base of the extended ECV.
This strategy is accomplished by infusion of approximately X mmol bicarbonate
(X= negative BE in mM multiplied by extended ECV). The extended ECV is
approximately 20% of the body weight in kg or l. Careful monitoring of
acid-base variables is necessary. Two errors are possible. Hours later, H+-ions
from the cells enter the extended ECV and a further bicarbonate infusion is
necessary. Correction of the primary disease (insulin to diabetic
ketoacidosis) may in itself cure the acidosis by combustion of keto-acids to
bicarbonate with the danger of overinfusion with bicarbonate.
Continuous
control of acid-base variables over days is therefore important.
Rapid
infusion of bicarbonate may be dangerous. A rapid decrease in [Ca2+]
releases tetanic cramps (see
alkalosis above). Administration of an overshoot of Na+-bicarbonate
leads to volume expansion, pulmonary oedema, and a new equilibrium with too
much CO2, which diffuses into the cells, worsening the intracellular
acidosis, and causing bicarbonate efflux accompanied by hyperkalaemia.
The final correction of a metabolic acidosis
is always renal.
4. Metabolic Alkalosis
is
caused by a primary accumulation of strong bases in the extended ECV. Both the
[bicarbonate] and the [non-carbonic buffer base] is increased, so the BE is
increased (Fig. 17-12). The actual [bicarbonate] is often above 27 mM, which
is the renal plasma concentration threshold for reabsorption. Above this
threshold lots of bicarbonate is lost in the urine.
Vomiting (loss
of gastric acid and volume depletion), increased metabolism of lactate and citrate (turns into bicarbonate and
water), and excessive intake of bases towards gastric ulcer can cause this form
of alkalosis. Long-term use of thiazides and loop diuretics, K+-deficiency,
and excess secretion of mineralocorticoid increase the H+-secretion
in exchange for Na+ in the distal tubules. This increases renal
bicarbonate reabsorption and leads to metabolic alkalosis.
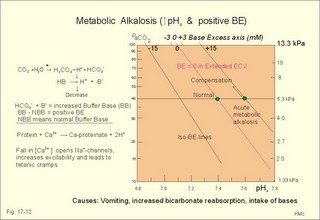
Fig. 17-12: Acute metabolic alkalosis: The base excess is increased. The
hypoventilatory compensation is shown with an arrow.
The hypoventilatory compensation
reduces pH, but raises PaCO2 (Fig. 17-12). The compensation is never total, since the rise in PaCO2 and the fall in PaO2 in
itself oppose the hypoventilation.
The delayed renal correction increases bicarbonate excretion by reducing
its reabsorption. This correction is also counteracted by the rise in PaCO2,
which stimulates tubular bicarbonate reabsorption. The final renal correction of metabolic disorders takes several days.
Careful
monitoring with replacement of Na+ and K+ is essential,
in order to improve the renal excretion of bicarbonate. In metabolic alkalosis
the kidneys increase H+-secretion less than the bicarbonate
filtration, whereby the bicarbonate excretion is increased. - Metabolic
alkalosis combined with hypokalaemia and reduced ECV often has increased H+-secretion, whereby the bicarbonate excretion is reduced.
Such cases must be treated with NaCl and KCl.
Only
rarely is it necessary to infuse acid, when deficits of NaCl, K+ and Mg 2+ are corrected.
A
patient with metabolic alkalosis is therefore rarely treated with infusion of
acids. In the very few cases, the acid of choice is an ammonium chloride solution, which produce H+, when NH3 is used for carbamide (urea) production in the liver. Metabolic alkalosis is
difficult to compensate by the body and difficult to treat. Each OH- has a molecular weight 17 times larger than H+, so OH- passes the membrane channels comparatively slowly in metabolic
alkalosis compared to the H+ -transfer of metabolic acidosis.
This results in delayed intracellular transfer and buffering of the alkalosis.
Cerebral insufficiency is common in alkalosis, and the respiratory centre is depressed. Alkalosis
displaces the oxyhaemoglobin dissociation curve to the left, impairing the
delivery of oxygen to the tissues. Dissociation of protein molecules may lead
to tetany. Protein anions bind Ca2+ during alkalosis, reduces the free serum [Ca2+] and triggers tetanic cramps (see explanation).
The nine van Slyke
conditions
These four primary acid-base disturbances
and their four compensated or chronic types constitute, together with the
normal condition, the nine van Slyke
conditions (Fig. 17-13). Plotting the measured pH and PCO2 in the acid-base chart allows estimation of the base
excess (BE), and combined with the case history, the correct diagnosis can be
reached.
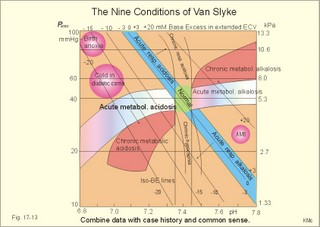
Fig. 17-13: The Siggaard-Andersen acid-base chart with the 9 conditions
of van Slyke (modified with permission).
Please
observe that each point on the chart can be reached in several ways. Van
Slyke, who made the first apparatus to diagnose acid-base disturbances,
emphasised the importance of the case history and common clinical sense. The
importance is illustrated by the following three examples. The first example
is a case of respiratory acidosis due to chronic obstructive lung disease (COLD in Fig. 17-13) and a metabolic
acidosis due to diabetic coma. Without the case history it is difficult to diagnose
the primary and secondary events in the development of the patients condition.
The second example is a case of respiratory
alkalosis due to acute mountain sickness (AMS), complicated by a metabolic
alkalosis (AMS in Fig. 17-13), because the patient is loosing acid by
vomiting. The third example is a serious case of birth anoxia with a combined
respiratory and metabolic acidosis. In this case instantaneous intubation and
oxygenation with 50-40-30 % oxygen saved the newborn.
The
case history, not the acid-base variables, is the only source to the sequence
of events.
High Altitude Acid-Base correction
At high altitude, the Van Slyke Equation has to be modified with a correction factor such that the acid-base charts are suitable. That way an acid-base correction is performed to the same altitude of residence and not to sea level values, that would be an agression instead of a return to baseline values. See Full text
Equations/Reactions (Eq)
· Acid (HB) is defined as a compound that can release a hydrogen ion or proton
(H+), whereas a base (B-)
can take up H+. The H+-concentration is symbolised as [H+]
just as other concentrations:
Eq 17-1: HB ® H+ + B-, where the dissociation constant (K´) is
defined by:
K´ = [H+]* [B-]/[HB].
· This is the mass
action equation, which can be logarithmically rearranged to:
Eq 17-2: pH = pK´ + log ([B-]/[HB]);
The pK´ is equal
to the pH, when the acid is 50% dissociated.
· For the carbonic acid system:
Eq 17-3: CO2 + H2O Û *H2CO3 Û H+ + HCO3-
Eq 17-4: pH = pK + log([bicarbonate]/[dissolved CO2]).
· This is the
Henderson-Hasselbalch-Equation - a logarithmic rearrangement of the mass
action equation.
· The law of Henry: Dissolved CO2 (ml l-1 of blood) is
in equilibrium with and equal to PCO2 multiplied by the solubility coefficient a according to Henrys law: [dissolved
CO2] = a* PCO2 (for
the definition and size of a for CO2 see Table 13-1):
Eq 17-5: (0.51 × 1000 × PCO2 )/(760 × 22.22) = (PCO2 × 0.03) mM
with PCO2 in mmHg..
One mmol of ideal gas equals 22.4 ml STPD and since CO2 deviates slightly from
an ideal gas its molar volume is only 22.22 l per mol. All the constants
combine to 0.03.
With kPa as unit for PCO2 (760 mmHg equals 101.3 kPa):
0.03 × 760/101.3 = 0.225; with this new constant the dissolved CO2 is equal to: (PCO2 × 0.225) mM.
· At normal ionic strength and temperature
the pK is 6.1
Eq 17-6: pH = 6.1 +
log([bicarbonate]/(0.03 × PCO2))
with PCO2 in mmHg or
pH = 6.1 + log([bicarbonate]/(0.225 × PCO2))
with PCO2 in kPa.
This (Eq 17-6) is the final Henderson-Hasselbalch-Equation.
· Proteins dissociate during alkalosis:
Eq 17-7: Protein + Ca2+ ® Ca-proteinates + 2 H+, and
the equilibrium dislocates towards the right. Of all the proton-binding groups
on proteins, the calcium-binding groups are only a minor fraction.
Self-Assessment
17. Multiple Choice
Questions
I. A patient who has been
vomiting for several days is in hospital with the following findings in a
sample of arterial blood: pH = 7.60; Base
Excess = +20 mM; and PaCO2 = 6.7 kPa (50 mmHg). Each of the
following disorders and statements has True/False options:
A. Compensated respiratory alkalosis.
B. Metabolic alkalosis
C. Hypoventilation
D. Increased base deficit
E. Increased bicarbonate concentration.
II. The following five statements have True/False options:
A. In connection with alkalosis the free serum [Ca2+] is reduced, as proteins liberate H+ and bind more Ca2+.
B. Metabolic acidosis is diagnosed by a negative base excess (base
deficit).
C. The titratable acid of the urine is the amount of acid needed to
titrate the acid urine to the pH of plasma.
D. The treatment of a patient with acute metabolic alkalosis and a base
excess of 20 mM is hypoventilation in a respirator.
E. Acute Mountain Sickness often causes
vomiting.
Case History
A.
A
female patient, 25 years of age, is undergoing a routine examination at an
outpatient ward. Her pH is 7.42, and PaCO2 is 5 kPa (37 mmHg).
The
patient is suffering from a disorder with acute attacks of anxiety, panic,
dyspnoea and tetanic cramps.
A
week later the patient is admitted to the emergency ward of the hospital with
severe hyperpnoea and tetanic cramps. She has abnormal blood gas tensions: PaCO2 2.7 kPa (20 mmHg), PaO2 14.6 kPa (110 mmHg), and pH is 7.64.
1. Read her Base
Excess of the extended ECV at the routine check by the help of Fig.
17-13.
2. Read her Base
Excess of extended ECV during emergency conditions by the help of Fig. 17-13.
3. What is the most
likely diagnosis?
Case History B
A
56-year-old male with chronic bronchitis and emphysema suddenly developed
respiratory failure during flying in a pressurised intercontinental plane. The
cabins of commercial aeroplanes are pressurised to a PB of 80 kPa
corresponding to 2000 m. The
patient was bluish in colour and the cabin personnel gave him a mask for
breathing with pure (100%)
oxygen, which was continued until
landing. The patient was then
admitted to hospital. An arterial blood sample (without supplementary oxygen)
revealed the following: PaO2 6.7 kPa (50 mmHg), PaCO2 8
kPa (60 mm Hg) and pHa 7.44. The saturated water vapour pressure at body temperature is 6.26 kPa.
1 Calculate PIO2 in the moist tracheal air of the patient,
when he breathes the air of the pressurised cabin just before respiratory
failure. Is the oxygen tension insufficient?
2 Calculate the PAO2 of the patient at the hospital assuming PACO2 to be equal to PaCO2 and RQ =1.
3. Read the base excess of the extended ECV by the help of Fig. 17-13 and
describe the acid-base disorder.
Case History C
Two
male persons with a normal arterial pH (pHa = 7.40) are in
hospital. One person starts to vomit excessively, which increases his pH to
7.80. The other person, a fit athlete, does a cardiopulmonary exercise test,
which reduces his pH to 7.00.
1. Calculate the three
concentrations of H+ (in nmol l-1 = 10-9 mol
l-1) at these conditions.
2. The two changes
in pH from the normal pH = 7.4 are similar. Why are the related changes in H+ concentration not identical?
3. Describe other
conditions with metabolic acidaemia and metabolic alkalaemia.
Try
to solve the problems before looking up the answers.
Highlights
· The
pH of the intra- and extra-cellular body fluids is maintained within narrow
limits by the co-ordinated function of the respiratory and the renal system.
· Persons
on a high-protein diet produce 50-100 mmol fixed or non-volatile acids and 24
mol of volatile carbonic acid
daily.
· Produced
and ingested acid-base is normally excreted, so the acid-base balance is
maintained.
· The
kidneys prevent loss of bicarbonate. Of the renally ultrafiltered bicarbonate
(4500 mmol daily) we reabsorb 90% already in the proximal tubules, and hardly
any bicarbonate is excreted in the urine.
· Urinary
buffers (ammonia and phosphate) are essential for the excretion mechanism of
non-volatile acid.
· Acidosis
(acidaemia) is defined as a disorder with pH in the arterial blood (pHa)
less than 7.35, and alkalosis (alkalaemia) is defined as a condition with pHa larger than 7.45.
· Respiratory
Acidosis is caused by hypoventilation (or breathing of CO2 containing air). Hypoventilation is associated with an impaired ability to
eliminate CO2, whereby PaCO2 increases and the
accumulated CO2 reduces the arterial pH.
· Respiratory
Alkalosis is caused by hyperventilation, which is disproportionately high
compared to the CO2 production, whereby the PaCO2 falls
and the pH increases.
· Metabolic
Acidosis is caused by accumulation of strong acids in the extended ECV.
Metabolic acidosis is diagnosed by negative base excess, because both types of
buffer bases are reduced.
· A
primary accumulation of strong bases in the extended ECV due to vomiting,
increased metabolism of lactate/citrate and excessive intake of bases cause
metabolic alkalosis.
· Respiratory
acid-base disorders are caused by primary changes of PCO2 , and are
compensated by altered renal excretion of acid in a matter of days.
· Metabolic
acid-base disorders are caused by primary changes in Base Excess, and are
compensated partially by the lungs in a matter of hours. The final correction
of metabolic disorders is always renal and takes several days, because
production and activation of cellular enzymes and hormones are involved.
· Alkalosis
dissociates protein molecules, which bind ionised calcium. The hypocalcaemia opens Na+ - channels, and the influx
increases the excitability of neuromuscular tissues, which releases tetanic
cramps.
Further Reading
Astrup,
P. and J.W. Severinghaus. "The history of blood gases, acids and
bases." Munksgaard, Copenhagen, 1986.
Koeppen,
B M. Renal regulation of acid-base balance. Am.
J. Physiol. 275 (Adv. Physiol. Educ. 20): S132-S141, 1998.
Siggaard-Andersen,
O. The acid-base status of the blood. Munksgaard,
Copenhagen, 1974.
Siggaard-Andersen,
O & OH Gøthgen (1995) Oxygen
and acid-base parameters of arterial and mixed venous blood. Relevant versus
redundant. Acta Anaesthesiol Scand 39.
Suppl 107, 21-27.
Siggaard-Andersen,
O and N Fogh-Andersen (1995) Base excess and buffer base (strong ion
difference) as measure of a non-respiratory acid-base disturbance. Acta
Anaesthesiol Scand 39. Suppl 107, 123-128.
Paulev PE, Zubieta-Calleja GR. Essentials in the diagnosis of acid-base disorders and their high altitude application.
J Physiol Pharmacol. 2005 Sep;56 Suppl 4:155-70 Full text
Return
to top
Return
to content
|